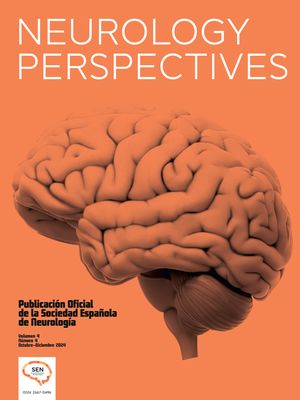
Biomaterial and stem cell -based treatment strategies for diseases of the central nervous system
Más datosBiomaterials are currently used in a wide range of applications in regenerative medicine, and to date, a large number of compounds have been designed as approach to different nervous system diseases.
Bioactive materials contain biologically active compounds such as enzymes, ceramics, drugs, antibiotics, vitamins, growth factors or stem cells that when conjugated with nanoparticles can promote an active response in the host tissue.
The present article carries out a systematic review and a critical analysis of the challenges that must be solved in the synthesis of biomaterials and in the evaluation of their therapeutic efficiency.
MethodologyThe present systematic review followed the PRISMA guidelines and included studies from PubMed Databases, published between 1997 and 2020.
ResultsThe search resulted in a total of 55 articles eligible for critical analysis. The available literature show that bioactive materials should exhibit two key properties:
1.The chemical–physical characteristics that give rise to the binding between the polymer and the active compound (biocompatibility) and
2.The scaffolds with a biologically active component must have the desired effect on the target within the host tissue.
ConclusionsThe use of bioactive scaffolds is an innovative and personalised approach to many nervous system diseases. The use of new Bioengineering strategies had increased the potential use of these tools in order to resolve daily clinical problems. More studies will be required to assess the efficiency of these strategies in clinical trials and to solve the potential challenges between host and bioactive scaffold response.
Los biomateriales tienen una gran aplicación en la medicina regenerativa y hasta la fecha se ha desarrollado una amplia variedad de compuestos para el tratamiento de diferentes enfermedades del sistema nervioso.
Los materiales bioactivos contienen compuestos biológicamente activos, como enzimas, cerámicas, fármacos, antibióticos, vitaminas, factores de crecimiento y células madre, los cuales, al combinarse con nanopartículas, son capaces de provocar una respuesta activa en el tejido del huésped.
Presentamos una revisión sistemática y un análisis crítico de los retos de la síntesis de biomateriales y la evaluación de su efectividad terapéutica.
MétodosEsta revisión sistemática sigue las recomendaciones de la declaración PRISMA; analizamos estudios incluidos en la base de datos PubMed publicados entre 1997 y 2020.
ResultadosUn total de 55 artículos cumplieron los criterios de inclusión. Según la evidencia disponible, los materiales bioactivos deben presentar dos cualidades principales:
1)mostrar características físico-químicas que permitan la unión entre el polímero y el compuesto activo (biocompatibilidad), y
2)los andamios bioactivos deben ejercer el efecto deseado en la diana del tejido del huésped.
ConclusiónEl uso de andamios bioactivos supone una aproximación novedosa y personalizada a muchas enfermedades neurológicas. Gracias al uso de la bioingeniería, el potencial de estas herramientas para resolver problemas de la práctica clínica ha ido aumentando. Se necesitan ensayos clínicos sobre la eficacia de estas estrategias, así como estudios que superen los retos que plantea la respuesta del huésped a los andamios bioactivos.
Novel therapeutic strategies are based on the development of new materials and technologies and aim to improve treatment efficiency. The development of biomaterials with a greater effect beyond their role as a physical barrier has recently seen several advances. However, there are still open challenges related to the design of such materials and their therapeutic efficacy based on the principles underlying their activity and their clinical impact.
Promotion of neural tissue regeneration in central and peripheral nervous systems is one of the main challenges in the development of efficient therapies for many diseases involving neural injury and cell death as the final pathological process due to mechanical, infectious, vascular and idiopathic aetiologies.1
Tissue regeneration strategies are normally divided into three categories:2
- 1.
Direct injection of bolus cells into the tissue of interest or into systematic circulation.
- 2.
Implantation of cells after they have been combined to form a three-dimensional tissue structure.
- 3.
Scaffold-based delivery of signalling molecules such as low-molecular-weight drugs, proteins and oligonucleotides that stimulate cell migration, growth and differentiation.
The use of scaffold-based delivery approaches has been one of the most successful strategies in pre-clinical studies in regenerative medicine, suggesting that spatio-temporal control over the location and bioactivity of the factors after their implantation into the body is essential for achieving a therapeutic effect. Bioactive compounds such as mitogens that stimulate cell division, growth factors that have proliferation-inducing effects and morphogens that control the generation of tissue form are some of the main signalling molecules used in scaffold-based delivery therapies.2
Rationally designed bioactive scaffolds are promising biotechnological therapy materials due to their capability to promote targeted tissue regeneration, and not only provide physical and biochemical support for regeneration but also create a favourable cell microenvironment. This will promote cell migration and targeted differentiation in order to regenerate the damaged tissue.3
Scaffolds can be designed with a wide range of morphological and mechanical characteristics according to the target tissue. One of the main goals in the synthesis of these materials is to emulate the local extracellular matrix (ECM) of the host microenvironment, and therefore promote the recruitment, differentiation, release and migration of cells.4 These biomimetic materials are generally designed using the covalent ligation of a peptide ligand from a native protein to a material to impart biological activity. The materials can be designed to interact with signalling molecules through the decoration of bioactive epitope sites for increased local concentration, stability and prolonged release. The challenge in this case is to create scaffolds with both the maximum number of epitopes and control of the epitope number, and the realisation of such scaffolds will be beneficial for the further development of various regenerative medicine approaches.5
To date, the efficacy of bioactive scaffolds has been analysed mostly in pre-clinical studies using animals and in-vitro models, and the actual efficacy of these therapeutic strategies in humans is unclear due to the lack of clinical trials.6 The main challenges for the design of these materials are related to the survival of the stem cells that are crucial for the reestablishment and regeneration of the injured tissue, pharmacology of the bioactive compound and the viability of the scaffold related to therapeutic efficacy, pharmacokinetics and pharmacodynamics, and control of the potential risks and secondary effects related to these methods.
Another perspective of innovation of bioactive scaffolds is related to the life cycle of cells called senescent as a therapeutic target, and the activation of mechanisms of “cellular resilience” that can improve their functional qualities beyond their usual lifetime. Interestingly, this is the case of adipose cell, that have been tested as precursors of fibroblasts and stem cells and which also represent the most important site of the accumulation of senescent cells in the body. Currently, modification of the cell cycle is considered to be key to preserving the body's vitality.7 The present systematic review carries out a critical analysis of these factors and explores the current trends in this field.
MethodologyA systematic review was performed under the criteria and flow diagram of the PRISMA Protocols (Fig. 1).
Study selection and inclusion and exclusion criteriaThe article inclusion to this review was determined by the following inclusion criteria:
- •
The manuscript should be written in English.
- •
The time of Publication should be until 2020.
- •
The syntaxis of the search should be: [nervous system] AND [scaffolds], [stem cells scaffolds], [bioengineering].
- •
The article should be indexed in one of the following data bases: Pubmed.
- •
The article selection included original articles, review articles, case reports and clinical trials.
The article exclusion to this review was determined by the following inclusion criteria:
- •
Articles in any other language as English.
- •
Articles without an Indexing in PubMed.
- •
Letter to editors or any other article type not included in the inclusion criteria.
In order to identify and eliminated duplicated articles, we imported the data to the Endnote X8 reference Manager Software. Then we performed a selection of articles based on the presence of the inclusion criteria in the title or abstracts of the articles. This process was executed by three independent researchers in a parallel search. In the extracted data, we included the name of the biomaterial, the bioactive effect, the experimental model (in the case of an experimental study), translational perspective in the clinic and the advantages and disadvantages of each approach.
Data synthesisThis qualitative systematic review shows the extracted data in the tables at the end of the manuscript each table expose the different approaches with the use of bioactive scaffolds and stem cells for diseases in central nervous system and peripheral nervous system. A more detailed overview of the biomaterial constitution, pharmacological kinetics and dynamics is presented in each sub-topic of this manuscript.
Survival of stem cellsStem cells are unspecialised cells with the capacity for continuous self-renewal and differentiation into a range of cell types. The recent efforts for the regeneration of neural stem cells (NSC) have been focused on biomaterials and bioengineering that emulate the NSC niche in order to establish an adequate microenvironment that promotes neurogenesis and differentiation. The biophysical signals and the release of biochemical factors are the main parameters that regulate NSC survival and differentiation.8
Nevertheless, because the NSC niche in adult mammalians has limited regenerative capabilities, the effective regeneration of the central nervous system (CNS) requires the reconstitution of its embryonic counterpart. During the early developmental phase, the radial glia (RG) is the main NSC that differentiates into neurons and glia. The RG encompass the whole CNS parenchyma and serves as a substrate for neural migration. During the early neurogenesis, blood vessels invade the CNS and interact with the NSCs, giving rise to a neurovascular niche. In the adult brain, the neurogenic RG can be recovered to some extent after a lesion, suggesting an endogenous attempt of reconstitution of the embryonic niche of NSC.9
Glycoproteins have been shown to play a major role in the migration of neural progenitors. In particular, tenascin-C contributes to the stem cell niche in the subventricular zone (SVZ) where it is highly expressed, and some of its main functions are changing the response to growth factors, enhancing sensitivity to FGF2 and decreasing sensitivity to BMP4 which promotes EGF receptor acquisition. A new role for endothelial cells and oligodendrocytes is key for such growth factor production. Many primary neurons such as cerebellar granule neurons, spinal motor neurons and dorsal root ganglion neurons have shown to exhibit increased average neurite lengths when they are cultured in-vitro on polyamide fibre coatings with tenascin-C.4
Platelet-derived growth factor-AA (PDGF-AA) is another potentially useful factor for in-vitro and in-vivo differentiation of neural stem/progenitor cells (NSPCs), and affects the expansion of NSPCs-derived oligodendrocyte population by acting as a survival, proliferation and differentiation factor. Thus, it is promising for use in conjunction with stem cell transplantation.10
Recent research advances have also shown that stem cell lineages other than NSCs can be used for treatment of CNS injuries, in particular the bone marrow mesenchymal stem cells (BMSCs). Some studies described the neural differentiation from BMSCs generated using butylated hydroxyanisole, noggin and retinoic acid. This approach opens new possibilities for the substitution of damaged neurons through so-called transdifferentiation.11
The peripheral nervous system (PNS) can partially recover from injuries caused by acute trauma and diseases such as diabetes mellitus. The nerve growth factor (NGF) is one of the main neurotropic factors for the survival, maturation and differentiation of sensory neurons. It is upregulated by dedifferentiated Schwann cells and has been used in biomimetic materials for neurite outgrowth.12
Pharmacology of the bioactive compounds and scaffold viabilityBioactive compounds such as growth factors are soluble-secreted signalling polypeptides that can induce variable cellular responses in a biological environment. This type of response can result in cell actions such as control of migration, differentiation or proliferation of a specific subset of cells and cell survival.13
These signalling polypeptides bind to specific transmembrane receptors on the target cells that ultimately elicit an integrated biological response. Growth factors differ from other oligo−/polypeptide molecules in that they exhibit short-range diffusion through the extracellular matrix and act locally due to their short half-lives and slow diffusion.2
Multiple factors such as the diffusion through ECMs, number of target cells, type of receptor and subsequent intracellular signal transduction, and external factors such as the degradation and binding in the ECM, control the effects of these polypeptides. These variables should be taken into account for the design of biomimetic scaffolds and give rise to some of the pharmacological challenges for the use of such scaffolds.3
Two major strategies have been developed for the delivery of signalling polypeptides in bioactive scaffolds2 (Fig. 2):
- •
Chemical immobilisation of the growth factor into or onto the matrix. This involves the affinity or chemical interactions between the growth-factor-containing polymer substrate and the surrounding tissue.
- •
Physical encapsulation of the growth factors in the delivery system. This strategy will achieve an in-demand release of the signalling molecules to the surrounding tissue.
Schematic representation of the two main tissue engineering bioactive scaffold strategies used to present bioactive compounds/signalling molecules (Growth factors, mitogens, morphogens) to host tissue in order to promote tissue regeneration. (A) Physical encapsulation of the growth factors in the delivery system. This strategy will achieve an in-demand release of the signalling molecules to the surrounding tissue. (B) Chemical immobilisation of the growth factor into or onto the matrix. This involves the affinity or chemical interactions between the growth-factor-containing polymer substrate and the surrounding tissue.
One of the most important signalling molecules used as bioactive compounds in regenerative CNS and PNS medicine are neurotropic factors (NF) that are promising compounds for the regeneration of nervous tissue and can be potentially used for the treatment of several diseases related to neuroinflammatory or neurodegenerative processes.14 However, generally, these compounds show either unfavourable pharmacokinetics, or a short half-life, or are unable to cross the blood–brain barrier (BBB). Local injections have shown limited results, and the continuous administration has short duration and requires the use of invasive techniques such as osmotic pumps and direct interstitial therapy as the delivery vehicle, or the use of implants, hindering its application in clinical practice.
The supply of a dynamic dose of substances is one of the main challenges for the use of bioactive scaffolds because the vehicle of the compound should be suitable to the local physiology, and adapt to pH, temperature and proteolysis. Therefore, nano-scaffolds are an excellent option for the delivery of bioactive compounds. Nano-scaffolds are synthetised as reticulated polymers, can be designed with a wide spectrum of morphological and mechanical characteristics, and can be bound to pre-designed molecules for their controlled release. In addition to the emulation of the ECM, the scaffolds can be designed for recruitment, differentiation, proliferation and release of cells. However, the creation of a scaffold system with a controlled and precise release of NF is likely difficult and impractical, and mostly involves the use of polymers that require complex synthesis, in-situ assembly with toxic co-solvents, or the use of external releasants.15
The use of precise chronometrical studies to measure the release of the bioactive compound and the cellular response is not practical and in some cases, these studies cannot be used for the systematic analysis of the performance of these materials. In such a case, ultimately it is necessary for the scaffolds to bind a bioactive compound to obtain biocompatibility with a reproducible morphology and a load of NF.
Another type of materials called self-assembling peptides (SAP) can spontaneously form scaffolds through intermolecular interactions such as the van der Waals and electrostatic interactions and hydrogen bonds. In particular, Arginine–Alanine–Aspartic Acid–Alanine (RADA) has a long history of use in cell cultures and tissue bioengineering and can emulate the local ECM structure. Specifically, in neural tissues, it has been used in the NSCs of rats, mice and humans. It has been demonstrated to be fully biocompatible with NSCs and with hippocampal cells.
The use of controlled release of pharmacological compounds by enzymes had been studied using different approaches such as nano-scaffolds, nanoparticles and hydrogels. Many pharmacological therapies have focused on neuroinflammatory diseases in which different types of proteases are secreted. For example, matrix-metallo-proteinases (MMP) can be designed for specific peptide sequences and adjusted for on-demand release. In particular, MMP-2 has many substrates and a variable activity and is secreted by the active glia during inflammation. Two excision peptides are potentially good for the controlled release of bioactive compounds: Glycine–Proline–Glutamine glycine + Isoleucine–Alanine–Serine–Glutamine (GPQG+IASQ, CP1) that has a high MMP-2 activity and Glycine Proline–Glutamine–Glycine + Proline–Alanine–Glycine–Glutamine (GPQG + PAGQ, CP2) with low MMP-2 activity. The incorporation of these peptides in RADA 4 hydrogels is relatively simple and reproducible because these molecules can be formed during the initial synthesis of peptides with predictable purity.
NF are fragile molecules that depend on their three-dimensional structures for bioactivity, and usually are 10–20 times larger than the SAP molecules. This problematic property can severely affect the trade-off between the function of the NF and the self-assembling capability of the RADA 4 scaffold. The use of peptide analogues (PA) can potentially resolve this challenge with the smaller size of PAs enabling the self-assembly of RADA 4.16
Two promising NF PAs have been investigated. The first is the brain-derived neurotrophic factor (BDNF) associated with the MVG (DP1) sequence, and known as Neuro-Pep-1 that stimulated the release of BDNF and Trkb in H19-7 cells in hippocampal tissue of rats, and has been demonstrated to improve the spatial learning and memory of adult rats. The second is the ciliary neurotropic factor (CNTF) associated with the DGGL (DP2) sequence and modified for its diffusion through the BBB that has been shown to promote neurogeny in the dentate circumvolution and synaptic plasticity in the rat brain.
To date, tissue bioengineering approaches based on the use of electrospun nanofibre mats have been shown to induce the axonal outgrowth and prompt tissue regeneration after a CNS injury. The porous structures of these electrospun nanofibre mats are biologically similar to the ECM and their correspondingly similar microenvironment is favourable for adhesion and cell proliferation. The physico-chemical characteristics of the nanofibres are useful for the regulation of critical physiological and biochemical activities of cells, enabling the nanofibre mats to function as scaffolds for adhesion and support of the cells cultivated ex-vivo as well as their use in the in-vivo setting.17,18
Blue-green microalgae spirulina has been considered to be a highly promising possible food source in the future and contains many bioactive compounds with therapeutic functions. It is expected that the bioactive molecules of spirulina that resemble the ECM can promote the microenvironment conditions for cultivated cells in nanofibre spheres, forming a complex matrix similar to the host tissue.17
Control of risks and secondary effectsECMs are essential structures that contain a wide variety of bioactive molecules that induce the growth and regeneration of tissues. The development of synthetic ECMs for tissue bioengineering is an active field of study. A wide variety of scaffolding materials based on synthetic and natural materials have been studied for application in neural tissue. However, achieving the desired biochemical and morphological characteristics through modification is difficult for many of these polymers. Generally, these scaffolds require an additional design modification, toxic co-solvents or a chemical trigger in order to maintain or form a suitable structure. One of the major problems in the application of these scaffolds is the lack of methods for their effective biodegradation. Because of this, these materials can potentially lead to chronic inflammation at the site of administration. It is also possible that the degradation products of these biomaterials will be toxic. It has been shown that the peptide derived from laminin isoleucine–lysine–valine–alanine–valine (IKVAV) inhibits the formation of glial scars and induces axonal outgrowth after spinal cord lesions. IKVAV was attached to RADA 4 in order to attenuate the potential glial response regulated by the host and therefore have a beneficial interaction.16
For materials such as polyglycolic acid (PGA) and polylactic acid, another potential risk that should be taken into account is the decrease of local pH induced by the degradation by-products such as lactic acid and glycolic acid. This effect has been shown to be detrimental to the surrounding cells and tissue.
Collagen nerve guidance channels have also been fabricated with good results. However, collagen is difficult to handle during suturing and is expensive; on the other hand, collagen denaturalisation produces gelatine, which is easier to manage and does not express antigenicity in physiological conditions.12
The use of hydrogels is an excellent strategy for minimising risks because they are usually inert to biological processes, are degradation-resistant, can be easily processed, withstand the heat of sterilisation without damage and can be synthetised in many shapes and forms.19
When the scaffolds are combined with treatment strategies such as stem cell therapy, an additional spectrum of risks must be considered and studied. Although stem cell therapy is a highly promising approach for the treatment of many CNS and PNS diseases, the true utility of these approaches in the clinical setting is still unclear because many challenges must still be overcome before large-scale clinical trials can be performed. The potential risks for cell therapy products (CTPs) are related to the inherent complexity and heterogeneous nature of CTPs. The most commonly used products such as pluripotent stem cells (hPSCs), human embryonic stem cells (hESCs) and human-induced pluripotent stem cells (hiPSCs) have inherent risks for potential tumorigenicity which is a key safety concern.20
Tumorigenicity risk has been primarily associated with hPSCs because these cell lines can form teratomas and other types of tumours in immunodeficient animals. This effect is related to several residual, undifferentiated hPSCs that persist in the final CTP, and can initiate tumour development in transplanted patients. It has been reported that other factors such as cell transformation and mutations in proto-oncogenes or tumour-suppressor genes, accumulation of genomic aberrations during the establishment of an hPSC line, or genomic instability following prolonged cell passage also may play an important role in tumour development. Due to these unexpected effects, safety protocols should be developed prior to the use of this therapy in clinical trials.8,20
In stem cell-based therapies for brain injury, the use of bone marrow mesenchymal stem cells (BMSCs) offers several advantages because BMSCs show a strong safety record in clinical trials, absence of tumorigenicity and ethical controversies, and relatively easy isolation and growth. Their potential use in brain injury treatment will be carried out through the induction of neural transdifferentiation.11
Due to the above-described possible side effects, awareness of the bioethical implications of these procedures is crucial, and in making therapeutic decisions, balance in the risk/benefit ratio to the patient must be sought at all times and harm to the patient must be avoided to ensure the safety and benefit for the patient.21,22
Use of bioactive scaffolds in the central nervous systemRegeneration of CNS in adult mammals is extremely limited after an injury, and moreover, several pathological process cause rapid damage of the surrounding nervous tissue accompanied by release of inflammatory mediators, loss of local blood vessels and a cellular immune response that ultimately leads to further axonal injury and cell death.11
Furthermore, the CNS presents other challenging characteristics that must be considered for the creations of biomaterials. The most important is the CNS segregation from the circulating blood by the BBB and the blood spinal cord barrier (BSCB). In normal physiological conditions, these barriers allow the passage of only a few molecules through the tight junctions between the extracellular membranes of endothelial cells and astrocytes. This condition complicates the intravenous delivery of drugs and other therapeutic molecules. For this reason, polymers that can be intrathecally placed show great potential for the delivery of therapeutics into the brain and the spinal cord.
Biomaterial scaffolds are a promising therapeutic approach because they can be used as cell and bioactive compound vehicles that promote the host tissue response and protection, emulating the ECM of the brain with bioactive signals. The concomitant use of stem-cell based therapies can also promote regeneration and neuroprotection. Here, we present a list of the main therapeutic approaches for CNS obtained from the survey of the literature (Table 1).
Bioactive biomaterials in the central nervous system.
Biomaterial | Bioactive effect | Experimental model | Translational perspective | Advantages | Disadvantages | Reference/Author |
---|---|---|---|---|---|---|
Mesenchymal stem cell-derived exosomes | Neurogenesis and cognitive function recovery | Mouse model | Alzheimer's disease | -Production of neurotrophins-Neuroregeneration and functional recovery | - No clinical studies yet to quantify the efficacy of this approach. | Reza-Zaldivar, et al. 2019.23 |
IKVAV-PA, VKIVA-PA self-assembled nanofibres. | Neural transdifferentiation of human BMSCs. | Human BMSCs culture on IKVAV-PA and VKIVA-PA gels and laminin PDL-coated surfaces. | Traumatic injuries in the central nervous system. | -Induction of neuroectodermal lineage commitment.-Self-assemble into supramolecular nanofibres. | -Significant decrease inviability without spreadingon the VKIVA-PA gel- No clinical studies yet to quantify the efficacy of this approach. | Ji, et al. 2018.11 |
Fibrous scaffolds and hydrogel matrices | Promote cell survive | In vivo 3D microenvironments | Ischemic stroke | -Emulation of ECMs | - No clinical studies yet to quantify the efficacy of this approach. | Tang et al. 2018.6 |
NT-3/fibroin complex | Decreased IBA-1 positive cells. | Canine spinal cord. | SCI | - Inhibits inflammation- Enhances nerve fibre regeneration- Improves motor function | - No clinical studies yet to determine the optimal concentration of NT-3 used for humans | Li, et al. 2018.24 |
Injectable hydrogels in combination with stem cells | Stem cell adhesion, proliferation | In situ | Ischaemic stroke | Site-specific stem cell biomolecule delivery | Lack of control, non-uniform cell distribution and low cell penetration capacity. | Gopalakrishnan, et al. 2018.25 |
Chitlac-THICK | Neural network reconstruction | Primary cultures of hippocampal neurons from rat pups | CNS injured systems | - Promote neuronal growth, differentiation, maturation and formation of synapses | - No clinical studies yet to quantify the efficacy of this approach. | Medelin, et al. 2018.26 |
PCL/iPSC-NSCs | - Differentiation into neural stem cells | Rat spinal cords | SCI regenerationCell transplantation | - Could promote motor function- Non-problems ethical | - No clinical studies yet to quantify the efficacy of this approach. | Zhou, et al. 2018.18 |
Fungal metabolites | -Scaffolds for the design and synthesis of new multi-target ligands | In vitro | Alzheimer's disease | -Antioxidant and anti-amyloidogenic activity. | - No clinical or animal models studies yet to quantify the efficacy of this approach. | Piemontese, et al. 2018.27 |
P(TMC-CL)/Ibuprofen | - Decrease on RhoA activation- Favours CD163+ cell recruitment- Increases βIII-tubulin and GFAP positive cells | Dorsal hemi section rat SCI sub-cultured on ND7/23 cells | SCI | - Contributes to resolution of the inflammatory process- Supports axonal growth (βIII-tubulin cells) in the lesion periphery and cellular infiltration | - The folding of an electrospun fibrous fabric impaired nerve regeneration. | Pires, et al. 2017.28 |
Cell interaction with extracellular matrix IKVAV peptide | Neuronal differentiation | In vivo | Traumatic brain injury | Promote axonal regeneration | Not effect on astrocyte proliferation. | Sahab et al. 2017.29 |
Tenascin-C mimetic peptide amphiphile nanofibre gel | Neurite outgrowth | P19 embryonal carcinoma cells | -Spinal cord injuries-Repopulation of dysfunctional areas of the brain. | -Self assembled nanofibre-Transition from a solution to gel upon exposure to physiological divalent cations. | - No clinical studies yet to quantify the efficacy of this approach. | Bern, et al. 2016.4 |
Injectable non-toxic silanised-hydroxypropyl methylcellulose hydrogel | Neuronal differentiation of grafted cells | 3D environment | Neuronal damage | Secretion of tissue repair factors of stem cells | - No clinical studies yet to quantify the efficacy of this approach. | Kandalam et al. 2016.30 |
(RADA)4-IKVAV-CP1-MVG | Neurite outgrowth | In vitro PC-12 cells | Neuroinflammatory and neurodegenerative conditions | - Neuroprotection- Neurogenesis | - No clinical studies yet to quantify the efficacy of this approach. | Koss, et al. 2016.16 |
3D multichannel/LN silk fibroin | Stimulate growth, development, and the extension of primary hippocampal neurons | Culture of primary hippocampal neurons in vitro, to then implant them in a SCI of rats | SCI regeneration | - Promotes axonal extension- Mediates cell migration- Neuronal adhesion promotion- Stimulates blood capillary formation | - No clinical studies yet to quantify the efficacy of this approach. | Zhang, et al. 2016.31 |
(RADA)4-GG-BMHP1 | - Axon regeneration and lamination- Less reactive asterocytes (GFAP expression) and gliosis- Motor neuron recovery | hEnSCs implanted into rats | Chronic SCI | - Improvement in motor function- Neural differentiation- Higher myelination | - No clinical studies yet to quantify the efficacy of this approach. | Tavakol, et al. 2016.32 |
Poly(ethylene glycol) hydrogel with Platelet-derived growth factor-AA | Differentiation of neural stem/progenitor cells (NSPCs) | Neural stem/ progenitor cells culture in foetal bovine serum | Spinal cord injury | NSPC oligodendrocyte differentiation | - No clinical studies yet to quantify the efficacy of this approach. | Elliot, et al. 2015.10 |
RADA16-FGL | Promoted SC-NSC proliferation and migration into the 3-D scaffold | SC-NSCs from neonatal rats | SCI regenerationTissue engineering | - Self-assemble into nanofibrous structure and support SC-NSC adhesion and survival | - No clinical studies yet to quantify the efficacy of this approach. | Wang, et al. 2015.33 |
Radially aligned electrospun PLA70/30 fibres release L-lactate | 3D organisation and supportive function of radial glia embryonic neural stem cells | Radial scaffolds implanted into cavities made in the postnatal mouse brain | Traumatic injuries in the central nervous system. | - Reactivation of embryonic neurogenic and angiogenic programs- Maintenance of pools of neuronal and glial progenitor cells | - Specific scaffolding topology.- Risk of induction of neuroglial tumours. | Alvarez, et al. 2014.9 |
Human mesenchymal stem cells | Neuronal differentiation | In vitro | Brain damage | Produce neuronal progenitor and mature neural cell markers | - No clinical studies yet to quantify the efficacy of this approach. | Okolicsanyi et al. 2014.34 |
Hydrogel | Neuronal differentiations | In vitro | In jury environment and scarring | Greater axonal infiltration, astrocyte migration and myelinated axon growth. | Determine the most relevant biological features, and size scale and determine how to create similar architectures within biomaterials. | Khaing et al., 2014.3 |
Transplantation of stem cell in the urinary bladder matrix | Cell differentiation | In rats | Traumatic brain injury | Reduce neuron tissue lossRepair damaged neural network | Previous studies shown a significant neuronal loss | Wang et al. 2013.35 |
NT-3/Chitosan | - Axon regeneration | Spinal cord of rats | SCI regeneration | - Restoration of locomotor function | - No clinical studies yet to quantify the efficacy of this approach. | Wang, et al. 2013.36 |
Electrospun PCL-Spirulina nanofibre mat | Improves the growth and metabolic activity of primary astrocytes | Rat primary astrocytes exposed to Spirulina extract | CNS injured systems | - Alleviated astrocyte metabolism without cytotoxicity.- Could reduce inflammation induced by gliosis | - Spirulina extract is rarely released from the PCL-Spirulina mat to the media within 24 h | Kim, et al. 2012.17 |
Extracellular matrix | Neuronal differentiation as well as neuronal outgrowth | PC-12 cells | Injury of the CNS | Rich in axonal growth inducer proteins. | - No clinical studies yet to quantify the efficacy of this approach. | Mammadov et al., 2012.37 |
Nerve growth factor coated -poly(2-hydroxyethyl methacrylate) hydrogel- | Enhancing of neuron survival and sprouting. | Dorsal root ganglion cultures | Interfacing SNC electronic devices | -Controlled local neurotrophin delivery | - No clinical studies yet to quantify the efficacy of this approach. | Jhaveri, et al., 2009.19 |
3D aragonite coralline matrices | Neuronal tissue restoration | Hippocampal (dentate gyrus) neurons and astrocytes of rat pups | Neuronal tissue development in 3D | - Proliferation of neuronal, astrocytes and other glial cells- Support mesenchymal stem cells differentiation into osteoblasts | - No clinical studies yet to quantify the efficacy of this approach. | Peretz, et al. 2007.38 |
NT-3 from fibrin-HBDS scaffolds | - Enhances the sprouting of CGRP, ChAT, 5-HT of the raphe spinal tract | Spinal cord of adult rats | SCI regeneration | - Neuronal fibre sprouting- Reduction of glial scar formation | - Motor function does not improve | Taylor, et al. 2006.39 |
IKVAV-PA | Promote neurite sprouting and to direct neurite growth | Murine neural progenitor cells | Neuroinflammatory and neurodegenerative conditions | -Rapid differentiation into neurons | - No clinical studies yet to quantify the efficacy of this approach. | Silva, et al. 2004.40 |
Hydrogels containing peptide or amino sugar sequences | Promotion of cellular adhesion and proliferation. | Rat brain and spinal cord | Injury of the CNS | -Promotion of cellular infiltration and angiogenesis. | -The astrocytes could express factors that inhibits the axonal growth. | Plant et al. 1997.41 |
CNS: central nervous system, SCI: spinal cord injury, IKVAV: C16V2A2E, VKIVA: C16V2A2E4 scramble sequence, PDL: poly-Dlysine, PA: peptide amphiphile, BMSCs: bone marrow mesenchymal stem cells. NGF-β: nerve growth factor β, PLA: poly-L/DL lactic acid, NSC: neural stem cell, PCL: Polycaprolactone, CP1: metalloproteinase 2 cleaved sequences, GPQG+IASQ, NT-3: neurotrophin-3, P(TMC-CL): poly(trimethylene carbonate-co-ε-caprolactone), BMHP1: bone marrow homing peptide, hEnSCs: Human endometrial-derived stromal cells, CGRP: peptidergic sensory fibres, ChAT: cholinergic alpha motor neurons, 5-HT: serotonergic neurons of the raphespinal tract, SC-NSCs: spinal cord-derived neural stem cells, 3-D: three-dimensional, RADA16-FGL: AcN-RADARADARADARADAGGEVYVVAENQQGKSKA-CONH2, LN: Laminin, iPSC-NSCs: induced pluripotent stem cells-derived neural stem cells.
In the CNS, laminin is a critical protein in ECM and is a major component of the basal lamina surrounding the brain blood vessels. It has functions related to neural stem cell expansion, migration and differentiation, and neural development, and enhances the growth of neurites. Supramolecular nanostructures of laminin mimetic peptide amphiphiles displaying the α1-chain laminin-derived epitope (IKVAV) are self-assembling nanofibres with properties for differentiation of neural progenitors into neurons and transdifferentiation of BMSCs into neuronal lineage.
In another approach, the controlled release of bioactive PDGF-AA was carried out with an injectable nanoparticle/hydrogel drug delivery system in poly(lactide-co-glycolide) nanoparticles. This compound can be potentially used in the treatment of spinal cord injuries due to its activity for NSPC oligodendrocyte differentiation.10
Currently, the field of electronic-brain interface shows great potential, with electronic devices interfacing with the brain used for applications such as monitoring or stimulation of brain areas. Some examples are the use of such devices as interfaces for robotic controlled movements, deep brain stimulators or cochlear implants. To make these devices biocompatible and to aid nerve regrowth, biomimetic materials have been used for the delivery of neurotrophins/anti-inflammatory drugs. The poly(2-hydroxyethyl methacrylate) (pHEMA) hydrogel-coated substrates used as a neurotrophin delivery matrix are very useful materials because they can be designed to possess mechanical properties similar to those of brain tissue. It was shown that the use of these substrates enhanced survival and sprouting of dorsal root ganglion cells in vitro.
Use of bioactive scaffolds in the peripheral nervous systemOne of the main challenges in the peripheral nervous system regeneration is the promotion of neural outgrowth and structural guidance of the traumatic nerve gap. An appropriate recovery process can be stimulated with a favourable microenvironment at the site of the injury. Thus, the use of a scaffold that emulates the biochemical signals and structural support of healthy tissue can be an appropriate strategy in this context. Currently used therapeutic strategies such as end-to-end suturing, nerve autografting and local delivery of NGF are only useful in a few select cases. In Table 2, we provide a list of the main therapeutic approaches for PNS assembled from the survey of the literature.
Bioactive biomaterials in the peripheral nervous system.
Biomaterial | Bioactive effect | Experimental model | Translational perspective | Advantages | Disadvantages | Reference/Author |
---|---|---|---|---|---|---|
Micropatterned biodegradable polyesters clicked with CQAASIKVAV | Promotion of neurite outgrowth. | In vitro PC-12 cells. | Peripheral nervous system injuries. | -Promotion of SC proliferation and NGF secretion.-Physical contact-guidance effect. | - No clinical studies yet to quantify the efficacy of this approach. | Zhang, et al. 201842 |
Bioactive peptide PA nanofibres with NGF-β. | Promotion of neurite outgrowth. | In vitro PC-12 cells. | Peripheral nervous system injuries. | -High-affinity epitopes for growth factors.-Support of the generation of neural connections. | - No clinical studies yet to quantify the efficacy of this approach. | Okur, et al. 2018.5 |
Spinal grafting of the human NSI-566 | Promote axonal sprouting | In rats and primate models | Spinal trauma | -safety and tolerability, the dose of NSC.No side effects identified | -No apparent evidence of remodelling and improvement. | Curtis, et al. 201843 |
Hydrogel derived from porcine decellularised nerve tissue | -Schwann cell proliferation support-Peripheral nerve regeneration | Rat sciatic nerve defect model | Peripheral nerve injury | -Low immunogenicity-Emulation of the natural structure of ECMs in peripheral nerves. | -Low availability, due to the dependence of donors. | Lin, et al. 2018.44 |
Polyacrylamide/graphene oxide/gelatine/sodium alginate (PAM/GO/Gel/SA) composite hydrogel | -Schwann cell proliferation support | Schwann cells culture. | Peripheral nerve injury | -Biocompatibility-Exchange of nutrients | -Low donor availability-Length of nerve gap-Possibility of postoperative dysfunction. | Zhao, et al. 2018.45 |
LN-PA/GaG-PA | Schwann cells populated new-born nerves | In rats | Peripheral nerve injury | -Successful renervation better regeneration | Incomplete renervation | Mammadov et al. 201614 |
PFTBA-OECs+fibrin/collagen-chitosan | Axonal regenerationIncreases oxygen supply | Sciatic nerve injury in rats | PNINeurological diseases | - Promotes nerve regeneration and functional recovery- Reinnervate muscles- Increases the survival of OECs | - No clinical studies yet to quantify the efficacy of this approach. | Zhu, et al. 201446 |
Tissue engineered nerve grafts | Regenerative microenvironment of the peripheral nervous system. | Animal models/ Clinical trial | -Nerve regeneration and functional recovery | -Angiogenesis and electric stimulation. | -Limited donors-The need of a second surgery and morbidities during the procedure-Biocompatibility problems. | Gu, et al. 2014.47 |
Chitosan microspheres into collagen-chitosan scaffolds for the controlled release of nerve growth factor | Regeneration of motor and sensitive nerves. | Sciatic nerve gap in rats. | Peripheral nerve injury | -Stabilisation of NGF bioactivity. | -The half-life of many of many growth factors is very short. | Zeng, et al. 2014.48 |
Bone marrow stromal cells | Nerve regeneration | In rats | Peripheral nerve injuries | Distributed uniformly in the lumens of endoneural tubesMulti. protrusive shapes. | Inconsistent nerve regeneration | Jia et al. 2012.49 |
Laminin modified linear ordered collagen scaffolds loaded with laminin-binding ciliary neurotrophic factor | To control the dispersion and misdirection of the regenerating nerve fibres, and spouting axons | Rat sciatic nerve transection model. | Nerve injury | -Laminin could induce cell spreading and myelination. Laminin could also affect neuronal behaviour, such as proliferation, migration, target recognition, neurite outgrowth, and central synaptic differentiation | Its application is limited by the expensive cost of periodic administration and serious side effects caused by the diffusion in body fluids. | Cao, et al. 2011.50 |
Fibre scaffolds of bioactive polysialic acid | Increase of the mechanical stability of the electrospun fibre scaffolds | In vitro cell tests with Schwan Cells | Peripheral nerve regeneration | -The production of this material is low cost.-Higher fibre conductivity morphology | - No clinical studies yet to quantify the efficacy of this approach. | Assmann et al. 2010.51 |
Collagen-binding NGF-B | Peripheral nerve regeneration | In rat s | Sciatic nerve crush injury | Favourable functional recoveryBetter regeneration in axonal regeneration | Rapid diffusion in body fluidsRequires periodic injection of NGF, expensive and excessive doses give rise to side effects | Sun et al. 2009.52 |
Collagen-terpolymer terpolymer of poly(N-isopropylacrylamide) (PNiPAAm) | Promotion of nerve regeneration | Dorsal root ganglia | Peripheral nerve regeneration | -Allows the diffusion of nutrients and tropic factors. | -Incapacity for repair long defects | Newman, et al. 2006.53 |
Nerve growth factor grafted tricalcium phosphate and gluataraldehyde cross-linking membranes | Peripheral nerve regeneration | Cultured Schwann cells | Nerve injury | -Novel biomaterial incorporation of Schwann cells and covalently immobilisedNerve growth factor | Absence of Schwann cell proliferation. | Chen, et al. 2004.12 |
Poly(L-lactic acid) porous conduits | Nerve tissue incorporation and proliferation of vascularisation. | Sprague Dawley rats | Peripheral nerve regeneration | -Biodegradability-Allows vascularisation and retain structural integrity. | -Low availability, due to the dependence of donors. | Evans, et al. 2000.54 |
PA: peptide amphiphile, NGF β: nerve growth factor β, PC12: pheochromocytoma 12 cells, SC: Schwann cells.
NGF is an important compound for the survival, maturation and differentiation of peripheral nerves, and after injury, the production of this growth factor is upregulated by Schwan cells. Therefore, it has a central role in nerve reconnection following axonal damage. NGF binds to its high affinity receptor TrkA that is expressed by the nerve-cells-activating pathways such MAPK, Akt and PKC.
The binding of this compound in a scaffold can only be achieved through high-affinity epitopes for the stability, increased local concentrations and prolonged release at the site of action. PA nanofibres showed high affinity binding of NGF and good cellular differentiation in a PC 12 cells in-vitro model. This binding was achieved due to its epitope sequence that is crucial for the growth factor affinity. The use of collagen binding epitopes for NGF has also been reported.5
Following nerve regeneration, non-degradable materials used as scaffolds may have deleterious effects due to infection or mechanical impingent. Therefore, biodegradable materials such as collagen may obtain more favourable results, particularly for the use of collagen gelatines. The use of crosslinking agents such as glutaraldehyde allows the modulation of the mechanico-chemical characteristics of gelatine films. NGF, glial cell line derived neurotrophic factor (GDNF) and neurotrophin (NT-3) have been shown to enhance regeneration across nerve gaps. In this case, the gelatine contains primary carboxyl groups on its chain backbones that endow the collagen gelatine with a capability to bind covalently to the amine groups of these neurotrophic molecules with an appropriate cross-linking agent.12
The use of the tenascin-C biomimetic materials has also been explored for the regeneration of spinal cord injuries, and a high expression of this glycoprotein was observed after spinal cord injuries and regions of axon ingrowth. Tenascin-C also plays an important role during brain development by providing guidance to neural progenitor cells. It has been used in self-assembling nanofibre gels formed by PAs, where it encapsulates and provides physical support to the cells. The self-assembly process is triggered upon exposure to the physiological divalent cations such as Ca2+ 4.
DiscussionThis review illustrates the state of the art and the directions for future advances in the field of bioactive scaffolds and their use with stem cell therapies for the treatment of SNC and PNC diseases. We have critically outlined the major challenges for the development of these therapeutics and the current trends in the research for their use in regenerative medicine. The presented tables offer a comprehensive and analytic comparison between the most representative studies and experimental models in the field, with each article described in terms of the main advantages and disadvantages, and the translational perspective of each approach.
Regenerative medicine is a promising field in medicine and future advances of this field in the treatment of CNS and PNS diseases are highly important due to the lack of success of the current therapies for many CNS and PNS conditions. These poor results are a consequence of many factors such as the complexity of the isolation of CNS from the general circulation resulting in problems for the delivery of therapeutics, and also due to the intrinsic limited capacity of the cells populations in CNS and PNS for proliferation and response after cell injury.
The main objective of the current biomimetic materials research is to create scaffolds that emulate the microenvironment of the host tissue. This goal can be accomplished not only by using three-dimensional support and materials with the correct mechanical properties,55 but also by the use of signalling molecules that promote cell migration, proliferation and differentiation through these materials.
Another crucial factor for these approaches is related to the survival of stem cell populations in the scaffold that can be implanted locally. Different types of stem cells have been used for this purpose in regenerative medicine, but the efficacy of this approach is still unclear, because many concerns had been raised regarding the use of pluripotent stem cells. This is mainly due to the complexity of their population and processes occurring during their differentiation and proliferation that can result in a malignant transformation of these cell lineages. Therefore, more research is necessary in order to fully understand the cellular and genetic mechanisms of stem cells, and safety regulation and profiles of these therapies should be standardised prior to large-scale clinical trials. Such research will provide better insight regarding the utility of these approaches in contemporary medicine.
One of the main challenges in the field of bioactive scaffolds is related to the affinity and pharmacology of the signalling molecules attached to the scaffolds. Through the use of the signalling molecules, the scaffold should be able to present the correct number of epitopes with high affinity for binding with the bioactive compound and at the same time, maintain the mechanical and physical properties for its biocompatibility in the surrounding host tissue. The same issue is also found for the case of the kinetics of the receptors in the cell membrane or intracytoplasmic injection that require a more specific molecular evaluation process. Neurotropic factors have been the most promising signal molecules for this effect, but the reproducibility, predictability and safety of the results in the therapeutic target have yet to be thoroughly elucidated.
Conclusions and future directionsCurrently, the use of bioactive scaffolds and stem cell therapy is a very promising and active field of research in regenerative medicine, and the use of this approach may play a major role in the future for the treatment of various CNS and PNS diseases for which current therapies have failed, or where physiological cell self-renewal processes are insufficient or inadequate. The current data and experimental models of these strategies have been tested mostly in preclinical research either using in-vitro or animal model studies. Many challenges should be addressed in the design of these novel therapies and the safety profiles of these materials and stem cells must be fully studied and understood before large-scale clinical trials can offer more evidence about the true efficacy of these approaches. Overall, these developments bring us closer to the expected realisation of personalised medicine based on highly specific therapeutic designs with fewer side effects.
Funding statementThis research did not receive any specific grant from funding agencies in the public, commercial, or not-for-profit sectors.
To Dayana Magaly García-Alatorre for the technical support.