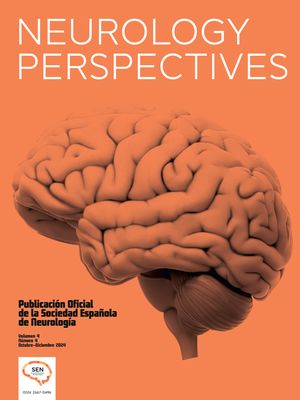
Biomaterial and stem cell -based treatment strategies for diseases of the central nervous system
More infoGlioblastoma (GBM), the most frequent type of brain tumour in adults, is highly aggressive and invasive. No curative treatment is currently available for GBM; patient survival is estimated at 15 months from diagnosis.
Background and objectivesLittle progress has been made in conventional treatment in over 20 years; this is largely due to the aggressiveness and localisation of the tumour and to the limited availability of systemic drugs that can penetrate the blood–brain barrier. In the search for new treatments for GBM, drugs showing promising results in preclinical studies frequently fail in phase I and II clinical trials due to the complexity and inaccessibility of the tumour, which is still not well characterised. This underscores the need to develop in vitro models mimicking specific characteristics of the blood–brain barrier and brain tissue, which may help to achieve higher success rates in later-phase trials. We evaluated the use of biomaterials for the development of complex preclinical models, such as three-dimensional models, which may play a crucial role in overcoming these obstacles.
ConclusionsThe applications and contributions of biomaterials in the study and treatment of GBM, from in vitro models to intelligent design of drug carriers, demonstrate the great potential of multidisciplinary management of such complex diseases as GBM.
El glioblastoma (GBM) es el tipo de cáncer cerebral más común en adultos, es altamente agresivo e invasivo. En la actualidad es considerado incurable, los pacientes presentan una sobrevida de 15 meses posterior al diagnóstico.
Antecedentes y objetivoLos tratamientos convencionales poco han cambiado desde hace más de 20 años, en gran medida debido a la agresividad del tumor, la localización y la limitada disponibilidad de tratamientos sistémicos con capacidad de rebasar la barrera hematoencefálica. En el desarrollo de terapias para GBM, se ha observado que fármacos con resultados alentadores en etapas preclínicas, frecuentemente fracasan en estudios clínicos de fases I y II, asociado a la complejidad y accesibilidad del tumor, que aún no está bien caracterizado y evidencia la necesidad de desarrollar modelos in vitro que emulen características específicas de la barrera hematoencefálica y del tejido cerebral en los que pueda evaluarse terapias con una tasa de éxito mayor en las posteriores etapas clínicas. En este artículo, se plantea que el uso de biomateriales en el desarrollo de modelos preclínicos complejos como modelos tridimensionales (3D), podrían desempeñar un papel crucial en la resolución de estos obstáculos.
ConclusionesLas aplicaciones y aportes de los biomateriales en el estudio y tratamiento del GBM abordados desde el desarrollo de modelos in vitro y el diseño inteligente de acarreadores de fármacos, demuestran el potencial del abordaje multidisciplinario en patologías complejas como el GBM.
Glioblastoma (GBM) is the most common and most aggressive type of brain tumour. It is more prevalent in men (male-to-female ratio of 1.6:1). The clinical manifestations of GBM are non-specific, and range from headache to personality changes. Diagnosis is based on biopsy results and imaging findings.1 Imaging studies are frequently performed at advanced stages since they are of limited value for differentiating between non-neoplastic lesions and early-stage GBM.2,3 The lack of effective diagnostic strategies and the limited understanding of the early stages of GBM result in treatment delays and fast disease progression. The mean survival time is estimated at 15 months. GBM is composed of multiple cell populations presenting high inter- and intratumoural genetic heterogeneity.4 This type of tumour almost exclusively affects the brain, although it may also involve the brainstem, cerebellum, and spinal cord, and presents a diffuse growth pattern. GBM may be a primary tumour, when it has no known precursor, or a secondary tumour, when a low-grade glioma (grade I or II) transforms into GBM (grade IV). Primary GBM is the most frequent type, accounting for approximately 90% of cases.5 Certain molecular patterns differentiate primary from secondary GBM; however, both are associated with activation of tumour suppressor p53 signalling pathways, the receptor tyrosine kinase/Ras/phosphoinositide 3-kinase signalling pathway, and the retinoblastoma pathway, enabling cells to proliferate uncontrollably and to escape from cell-cycle checkpoints.6
Standard treatment starts with maximal safe resection (if tumour extension and localisation allow it), followed by chemotherapy and radiotherapy. However, outcomes continue to be discouraging, with a 2-year survival rate of 18% after radiotherapy and chemotherapy with temozolomide, compared to 4% in patients receiving radiotherapy only; this is probably explained by the heterogeneity of GBM, which favours treatment resistance.7,8 New therapies for GBM showing favourable results in preclinical studies often fail in phase II clinical trials. This is the case for combined treatment with the epidermal growth factor receptor (EGFR) inhibitor erlotinib, and sirolimus, a mammalian target of rapamycin kinase inhibitor.9 Pilot studies of this combination therapy have reported a partial response rate of 19%, whereas phase I/II studies report no improvement.9
A deeper understanding of the pathophysiological mechanisms of the tumour and nearby structures involved in drug delivery may help to determine the therapeutic potential of a drug in preclinical studies using models that replicate the tumour microenvironment, thus preventing failure in later-phase trials.10
Biomaterials play a fundamental role in current medical research due to their biological, chemical, physical, and mechanical properties. These substances are biocompatible with biological systems and can therefore be used in various branches of biomedical research. The use of biomaterials in the development of three-dimensional (3D) cultures is a novel strategy for research into the pathogenesis of GBM and for testing new drugs. Furthermore, and with a view to developing more precise and effective treatments, biomaterials constitute a potential platform for targeted drug delivery, reducing adverse reactions, clearance, and the accumulation in different organs, compared to chemotherapy. These materials can also be used in such neurodegenerative diseases as Parkinson's disease and Alzheimer disease.11
BiomaterialsBiomaterials are compounds whose chemical, mechanical, physical, and biological properties make them compatible with biological systems.12 They can be optimised for specific purposes and biological environments. For example, they may be used to direct the course of a therapeutic or diagnostic procedure through interaction with the living organism.13
At present, biomaterials have generated particular interest in the field of biomedicine due to their multiple applications in health research, including the manufacture of medical devices, prosthetic devices, implants, and drug release systems. Furthermore, they are increasingly used for in vitro diagnosis of a number of diseases.14
Biomaterials may be classified as polymeric, metallic, ceramic, and composites (Fig. 1).15 Polymers are the most extensively studied biomaterials in clinical research. Polymeric biomaterials are further classified as natural or synthetic. The properties of synthetic polymers can be adapted to a wide range of applications; these materials are mainly used for implantable medical devices.16 Natural polymers resemble the extracellular matrix (ECM) of tissues and are therefore mainly used for tissue engineering and regenerative medicine.17
Metallic biomaterials are corrosion-resistant, conferring excellent long-term stability and mechanical resistance, making them ideal for manufacturing implants for tissue replacement.15,18
The key properties of ceramic biomaterials are high resistance to corrosion and temperature, fragility, hardness, and electric insulation. Therefore, they are mainly used as implants and in the repair and reconstruction of bones, joints, and teeth; they may also be used in the regeneration of hard and soft tissues.15,19
Current strategies in medical research focus on the development of biomimetic materials. These materials are designed to replicate the composition, structure, and mechanical properties of tissue in vivo,20 and can therefore interact with nearby tissue and prompt specific cell responses.15,21 In the healthcare industry, biomimetic materials are used for tissue engineering, regenerative medicine, as biosensors, and for drug delivery. For instance, hydrogels have been used to model the characteristics of brain tissue.22
Biomaterials as a tool for the study of tumours and for drug testingThe ECM is the non-cellular component that provides a physical scaffold for all tissues and organs. It plays an essential role in biochemical and biomechanical signalling, and therefore in tissue morphogenesis, differentiation, and homeostasis.23 The ECM accounts for approximately 20% of the total volume of the brain and occupies the space between neurons and glial cells.24,25 The composition of the brain ECM is different from that of other tissues as it contains few fibrous proteins and large amounts of proteoglycans, glycosaminoglycans (mainly hyaluronic acid [HA]), and glycoproteins (tenascin C and vitronectin).26
The ECM of a brain tumour presents high levels of collagen and HA; in this microenvironment, cells overexpress HA receptor CD44 and secrete such enzymes as metalloproteinases and hyaluronidases (Fig. 2), which degrade the ECM and promote tumour invasion in the brain.27 Alterations in ECM molecules and their complementary receptors therefore play an important role in the development and progression of gliomas.28,29 They have also been reported to contribute to drug resistance in several types of tumours.
Different biomaterials have been used for the development of 3D models of the ECM in GBM (Table 1).30 Due to the composition of the ECM in brain tissue (Fig. 2), HA has been widely used in these models, as well as other natural polymers, including gelatin, collagen,31 alginate,32,33 chitosan,34 polyacrylamide,35 and such synthetic polymers as polyethylene glycol (PEG).36,37 These biomaterials have been used alone and in combination, and combined with proteins, RGD peptides, or matrix metalloproteinase–cleavable peptides. Three-dimensional models of the ECM in GBM have been used to study cell migration, invasion, and interactions, and for drug evaluation.
Biomaterials used in the development of 3D models of glioblastoma.
Biomaterial | 3D model | Contributions | Reference |
---|---|---|---|
Hyaluronic acid | Hydrogel | HA-CD44 and integrin-RGD interactions promote chemoresistance. Hydrogels with higher HA content protected glioblastoma cells against chemotherapy. Similar findings have been reported in the clinical setting, where increased HA expression is positively correlated with tumour aggression. | 44 |
Collagen–hyaluronic acid | Hydrogel | Evaluation of combinations of HA with different types of collagen (type I/III and IV).Cell morphology was influenced by collagen type. Cell propagation and migration were dependent on HA concentration. These results suggest that GBM cells are sensitive to ECM-mimetic biomaterials. | 39 |
Chitosan and hyaluronic acid | Scaffolds | Scaffold-grown cells exhibited features of cancer stem cells, such as expression of genes that mediate epithelial–mesenchymal transition. Furthermore, they presented an undifferentiated phenotype and displayed greater resistance to drugs than monolayer cell models. This model mimics tumour behaviour and may be useful in basic research and preclinical studies. | 42 |
Hyaluronic acid and methacrylated gelatin | Hydrogel | Evaluation of HA of different molecular weights (10, 60, and 500 kDA) in methacrylated gelatin hydrogels. HA molecular weight had an impact on migration of GBM cells. These results may be useful in research into new targeted therapies. | 38 |
Methacrylated gelatin and gelatin | Bioprinting | Study of interactions between GBM cells and glioblastoma-associated macrophages, and evaluation of drugs aimed at inhibiting these interactions. The 3D models showed that glioblastoma-associated macrophages induce glioblastoma progression and invasion. Drugs inhibiting the interaction between the tumour and macrophages reduce tumour growth. | 43 |
Polyethylene glycol and hyaluronic acid | Hydrogel | Study of the effect of ECM stiffness on proliferation, dissemination, and gene expression of glioblastoma cells. Evaluation of matrices with 2 different grades of stiffness, mimicking the stiffness of normal brain and the stiffness of GBM tissue. Results suggest that changes in ECM stiffness in tumours play a major role in modulating tumour progression. | 37 |
Gelatin, alginate, fibrinogen | Bioprinting | Construction of a vascularised tumour by seeding spheroids onto a bioprinted blood vessel layer. The study investigated blood vessel marker expression and tested drug efficacy. This bioprinted model mimics the tumour microenvironment and is useful for understanding tumour biology and for in vitro drug testing. | 45 |
Chitosan–alginate | Scaffolds | Use of a chitosan–alginate scaffold and matrigel to evaluate secretion of factors promoting tumour malignancy (VEGF, metalloproteinase 2, fibronectin, laminin) in a glioblastoma cell culture. Scaffolds were subsequently implanted into nude mice to evaluate tumour growth and blood vessel recruitment. Results show that chitosan–alginate scaffolds promote the formation of a more malignant GBM phenotype than in monolayer or Matrigel culture conditions. This 3D model mimics the microenvironment of glioma cells and may constitute an effective platform for the development of treatments for GBM. | 46 |
ECM: extracellular matrix; GBM: glioblastoma; HA: hyaluronic acid; VEGF: vascular endothelial growth factor.
The use of biomaterials in 3D cultures enables the study and control of individual aspects of a complex ECM. Furthermore, the physical and chemical properties of biomaterials may be adapted to mimic specific characteristics of the ECM. Chen et al.38 used a hydrogel containing HA and methacrylated gelatin to evaluate the effect of HA concentration and molecular weight on the invasive phenotype of a patient-derived GBM specimen, and found that GBM cell migration was influenced by HA molecular weight. Rao et al.39 used a collagen–hyaluronan composite hydrogel to study cell morphology, propagation, and migration in a 3D culture, and found that the morphology of GBM cells was influenced by the type of collagen and that cell migration was inversely dependent on HA concentration. Wang et al.37 developed a PEG-based hydrogel to study the effects of ECM stiffness on GBM cell fate. Their results suggest that changes in ECM stiffness alter GBM cell proliferation, morphology, and migration in 3D cultures. These studies show that GBM cells are sensitive to ECM composition and that ECM dysregulation and stiffness influence the progression of GBM. These studies also analyse individual characteristics of the tumour microenvironment, enabling the identification of potential therapeutic targets.
Tumour maintenance and growth largely depend on the microenvironment. The tumour microenvironment comprises several types of cells and extracellular components, such as the ECM, growth factors, cytokines, and hormones, which surround the tumour promoting angiogenesis.37 The microenvironment of GBM has been modelled in 3D cultures, which have been used to study hypoxia,40 stem cell behaviour,41,42 interactions with multiple cell types,43 and immune cell infiltration.40 The use of 3D models has shown that an abnormal microenvironment not only promotes tumour growth but also modulates treatment response. Future research into new treatments for GBM should therefore use in vitro models that account for the role of tumour microenvironment in tumour progression, cell invasion, and drug resistance. The above underscores the importance of using biomaterials in the development of 3D models to obtain reliable data on treatment efficacy and results that can be extrapolated to clinical trials.
Drug delivery biomaterials: An alternative to glioblastoma treatmentNo curative treatment currently exists for GBM. Standard treatment has evolved very little over the past 10 years, with surgery being the initial treatment of choice. However, the diffuse growth of the tumour prevents complete resection of the cancerous tissue; therefore, patients must also undergo radiotherapy and alkylating chemotherapy with temozolomide after surgery.47 Wang et al.48 reported that radiotherapy with adjuvant temozolomide achieves substantial improvements in overall survival as compared to radiotherapy alone. Unfortunately, life expectancy in these patients continues to be poor. In recent years, studies have evaluated the use of such drugs as bevacizumab as an adjuvant to standard treatment with radiotherapy and temozolomide; this approach has achieved improvements in progression-free survival but has demonstrated no effect on overall survival.49 Although the different therapeutic approaches are associated with tumour recurrence 1–2 years after diagnosis, no standard treatment has been developed for tumour recurrence and little evidence is available on interventions that effectively increase overall survival.49,50
The limited effectiveness of the available therapies for GBM underscores the urgent need to develop new treatments. Further research should be conducted to address critical points related to the failure of these treatments, such as the blood–brain barrier, which restricts the passage of therapeutic agents (including small molecules and antibodies) into the central nervous system,51 or tumour heterogeneity, given the great molecular, genetic, and cellular diversity associated with chemoresistance.8
Biomaterials constitute a novel approach to drug delivery in GBM. The most frequently used materials are such polymers as poly lactic-co-glycolic acid (PLGA), whose biocompatibility is well documented and whose main advantage is the ease of controlling its degradation and, consequently, administration of the drug.52 PEG is used to reduce the tendency of particles to aggregate in physiological conditions. The reaction is pH-dependent; pH must be near or above the amino acid residue's pKa, forming covalent bonds that improve the solubility of drugs, liposomes, and nanocarriers, extending the drug's plasma half-life.53,54 Poly(glycolide-ɛ-caprolactone) is miscible with a wide range of polymers.55 Chitosan is a cationic polysaccharide used for drug delivery due to its abundant amine groups.56 Hyaluronic acid is a natural polysaccharide presenting good biocompatibility and degradability that can specifically bind to multiple cell surface receptors.57 These biomaterials have been used as carriers for different drugs, proteins, and antibodies in targeted drug delivery (Fig. 2).
Drug repurposingDrug repurposing is a safe approach to GBM treatment due to the pre-existing knowledge on the dosage, safety, and secondary effects of a given drug; this has a direct impact on research costs and drug development times.58 Phase I and II clinical trials have evaluated the antineoplastic effects of different drugs on GBM.59 For example, the antidiabetic drug metformin has been demonstrated to improve treatment outcomes in patients with prostate cancer.60 Likewise, studies are currently underway into hydroxyurea,61 gallium maltolate,62 rapamycin,63 chloroquine,64 and chlorpromazine.58,65
One promising approach to GBM treatment is the use of US Food and Drug Administration-approved chemotherapies with devices designed for local drug delivery; this route bypasses the blood–brain barrier, ensuring drug bioavailability while minimising adverse effects and the toxicity associated with systemic therapy.66,67 Furthermore, recurrent glioblastoma usually appears within 2 cm of the area of tumour resection. Local treatment may therefore inhibit recurrence, improving patient survival and making local administration a promising route for GBM treatment.
Marketed under the brand name Gliadel in the 1990s, a biodegradable polymer wafer loaded with the antineoplastic drug carmustine was approved by the US Food and Drug Administration for the local treatment of GBM. Gliadel represented the first clinical application of polymers for brain tumours.68 However, it is not included in current treatment regimes as its efficacy is not well established. Some factors associated with its lack of efficacy are directly linked to the pharmacokinetics of carmustine: it presents poor diffusion due to the stiffness of the polymer wafer, which prevents close contact with the edges of the resection cavity and results in wafer deposition at the bottom of the cavity.69 However, approval of this drug led the way to an important line of research in the treatment of GBM, with the publication of many studies into biomaterials for local drug delivery, including mouldable polymer matrices,70 microfibre implants,66 foams,67 microparticles,71 microspheres,72 conjugates,73 dendrimers,74 hydrogels,75 micelles,76 and patches.55
Rahman et al.70 developed a sustained-release system for multiple chemotherapeutic agents, including trichostatin A, etoposide, and methotrexate, for the treatment of GBM. The system was a mouldable PLGA/PEG polymer, which is biocompatible with vascular endothelial cells and U87-MG tumour cells. This system was designed for intracavitary administration and represented a novel delivery route for multiple drugs. Furthermore, its malleability enables adaptation to each patient, ensuring bioavailability of the chemotherapeutic agents in the area of greatest tumour recurrence. In line with this approach, more complex systems have been developed to manufacture soft, flexible patches or hydrogels with the mechanical characteristics of the native tissue, preventing mismatches between the implant and the brain.77 According to Jaworska et al.,55 drug delivery with patches can be regulated by their thickness and the area covered, which makes patches another option for controlled drug release. These studies demonstrate that biomaterials may play a crucial role in overcoming the limitations of current treatments for GBM due to their versatility from a biological, physical, and mechanical viewpoint, which minimises the adverse effects of drug-delivery systems. Furthermore, the use of biomaterials may increase the efficacy of future treatments, as other administration routes are unable to achieve therapeutic drug concentrations.
Some studies achieving favourable results in preclinical stages have moved forward to clinical trials exploring the introduction of antineoplastic drugs initially developed for breast, intestinal, skin, stomach, oesophageal, ovarian, or colon cancer. Table 2 summarises the biomaterials used as drug-delivery systems in clinical trials.78
Pilot studies and phase I/II clinical trials of biomaterials for drug delivery.
Phase | Biomaterial | Drug | Results | Reference |
---|---|---|---|---|
Pilot study | PLGA microspheres | 5-Fluorouracil (70 mg and 132 mg) | The overall median survival time was 98 weeks from the time of implantation. Two patients achieved disease remission. | 79 |
Phase I | 5-Fluorouracil (132 mg) | The median overall survival was 40 weeks. Two patients presented longer survival times. | 80 | |
Phase II | 5-Fluorouracil (130 mg) | The overall survival was 15.2 months in patients in the intervention group, compared to 13.5 months in the control group. | 81 | |
NCT00479765 Phase I/II | OncoGel™ | Paclitaxel (dose not specified) | The trial was terminated by the sponsor for commercial reasons. | 82 |
Pilot study | CuboSphere™ | Paclitaxel/carboplatin (≥15 mg) | Intracavitary chemotherapy in recurrent glioblastoma is feasible and safe. | 83 |
NCT02433392 Phase I | Biodegradable hydrogel microspheres | Irinotecan hydrochloride (≤75 mg) | The trial was terminated due to recruitment problems. Results not published | 84 |
Pilot study | 6-Carboxylcellulose | Cisplatin (45 mg) | Significant increase in median overall survival time as compared to the control group (surgery + radiotherapy). The dose was well tolerated and effective. | 85 |
NCT01526837 Phase I | Collagen sponge | Bevacizumab (0.25–25 mg/mL) | The trial was terminated by the researchers. Results not published | 86 |
In patients with GBM, the blood–brain barrier is disrupted due to cancer cell infiltration, which alters the structure and permeability of blood vessels throughout the course of the disease. This favours the entry of molecules of different sizes. Several studies have aimed to determine the optimum size of nanoparticles for delivery across the blood–brain barrier. In an in vitro study conducted by Hobbs et al., the critical pore size for intracranial human primary glioblastoma cell line U87MG was established at 7–100 nm.87 Betzer et al.88 reported an optimum size of 20 nm in an in vivo study with Balb/C mice, while Shilo et al.89 suggest a particle size of 20 nm based on the results of an in vitro study.
Tailored particle size, easy surface modification, improved solubility, multifunctionality, reduced toxicity, and improved pharmacokinetic and pharmacodynamic properties make nanoparticles an attractive delivery system for drugs targeted at brain tumours. This is the case with encapsulated and pegylated doxorubicin: these two presentations delay renal clearance, increasing half-life by 5.5% as compared to free doxorubicin. With this in mind, researchers have developed lipid, polymeric, silica-coated, metallic, and magnetic nanoparticles, which can be loaded with a wide range of active ingredients, including chemotherapeutic agents, anti-angiogenic compounds, sensitisers, tumour-specific targeting molecules, and contrast agents for detection.90 Fang et al.56 developed GBM cell-targeted nanoparticles with chlorotoxin, a peptide that recognises a wide range of neuroectodermal tumours. Nanoparticles were loaded with the alkylating drug temozolomide, demonstrating sustained stability and efficacy in drug-resistant cells. These approaches enable the administration of effective doses of chemotherapeutic agents to tumour cells, minimising toxicity in healthy tissue.56 They may also be coupled to radioactive compounds and traced in the body, with potential use in the diagnosis and monitoring of GBM.91 The versatility of nanoparticles in terms of structure, action mechanism, and administration route makes them an interesting alternative in the treatment of GBM as they may boost the efficacy of the loaded pharmacological agent (Fig. 3).
ImmunotherapyImmunotherapy for cancer is a promising treatment approach that aims to deliver targeted therapeutic agents in a precise manner. In GBM, immunotherapy research has traditionally focused on such cytokines as interleukin 2, antibodies including the VEGF inhibitor bevacizumab, inhibition of such checkpoints as PD-L1, tumour antigen vaccines (Rindopepimut), and chimeric antigen receptor (CAR) T-cell therapies. Interleukin 2 has been approved for the treatment of metastatic renal cell carcinoma and metastatic melanoma; however, it presents limitations due to its high dose-dependent toxicity.92 Bevacizumab binds and neutralises VEGF, and has been used in the treatment of colon, breast, and kidney cancer and glioma. The efficacy of PD-L1 inhibition is unpredictable in GBM due to specific molecular alterations, such as PTEN mutations.93 The Rindopepimut vaccine eliminates cells expressing epithelial growth factor receptor variant III (EGFRvIII); clinical trials have shown that its long-term efficacy is limited by the outgrowth of cells not expressing EGFRvIII.94 CAR T-cell therapies have been used successfully in the treatment of B-cell lymphoma, and their application is being studied in the context of GBM, with a wide range of tumour antigens, including interleukin-13 receptor alpha 2, EGFRvIII, human epidermal growth factor 2, erythropoietin-producing hepatocellular carcinoma A2, ganglioside 2, CLTX, B7-H3, CD70, and CD133. CAR T-cell therapy has achieved favourable results in preclinical studies; however, due to the limited efficacy observed in clinical trials, there is a need for further research and advances in immunotherapy.95 Immunotherapy may greatly benefit from new advances in administration technology and materials.96 The limitations associated with the short half-life of labile molecules can be solved with liposome or micelle encapsulation, as these formulations have demonstrated prolonged circulation times in the blood, improved efficacy, and fewer adverse reactions. Furthermore, the limited efficacy of some therapies is linked to their specificity, while tumour heterogeneity promotes immune evasion. Therefore, strategies have been developed to deliver treatments to multiple targets using nanoparticles loaded with antibodies, antibody fractions, cytokines, or pharmacological agents.97
ConclusionsGBM is highly invasive and is associated with poor prognosis and high recurrence and mortality rates. This is aggravated by the limited understanding of the initial stages of this type of tumour, which results in delayed diagnosis and treatment. In the search for treatments to improve survival times in patients with GBM, biomaterials provide a promising alternative for drug delivery, improving bioavailability and reducing adverse reactions to antineoplastic drugs. Biomaterials can also be used for local administration of therapies targeting tumour cells through controlled-release systems. Biomaterials have been shown to mimic the tumour microenvironment in vitro; these models enable the study of key features of tumourigenesis, cell proliferation, and drug resistance, both alone and as part of a single phenomenon, allowing more accurate descriptions of the pathophysiology of GBM. These materials have also been used to study cell–ECM interactions and as models of heterogeneous tumour microenvironments, enabling the study of molecular targets with new bioactive compounds. This approach has also led to the development of such novel disciplines as theranostics, where biomaterials, nanocarriers, and novel compounds converge in complex 3D models aimed at deepening our understanding of tumours and developing more efficient treatments.
Biomaterials are versatile, adaptable, and biocompatible compounds. Multidisciplinary research integrating methods from such areas as materials chemistry, biomedicine, bioengineering, and precision medicine will improve the reproducibility, stability, and characterisation of biomaterials, and enable the study of the pharmacokinetic and pharmacodynamic features of nanoparticles with a view to standardising validation protocols in preclinical and clinical trials. In summary, biomaterials constitute a promising tool in multiple biomedical fields as well as in the understanding and development of new therapies, not only for such tumours as GBM but also for other neurodegenerative diseases.
FinanciamientoEste artículo no recibió financiamiento.
AgradecimientosJanette del Rocio Aguilera Márquez y Guadalupe Tonantzin de Dios Figueroa agradecen la beca otorgada por el Consejo Nacional de Ciencia y Tecnología (CONACYT) durante el desarrollo de la Maestría en Ciencias.
Conflictos de InterésLos autores declaran que no tienen ningún conflicto de interés.
Ethical responsibilityThe authors declare that ethical responsibilities not apply to this kind of manuscript (review).
Declaration of interestsThe authors declare that they have no known competing financial interests or personal relationships that could have appeared to influence the work reported in this paper.