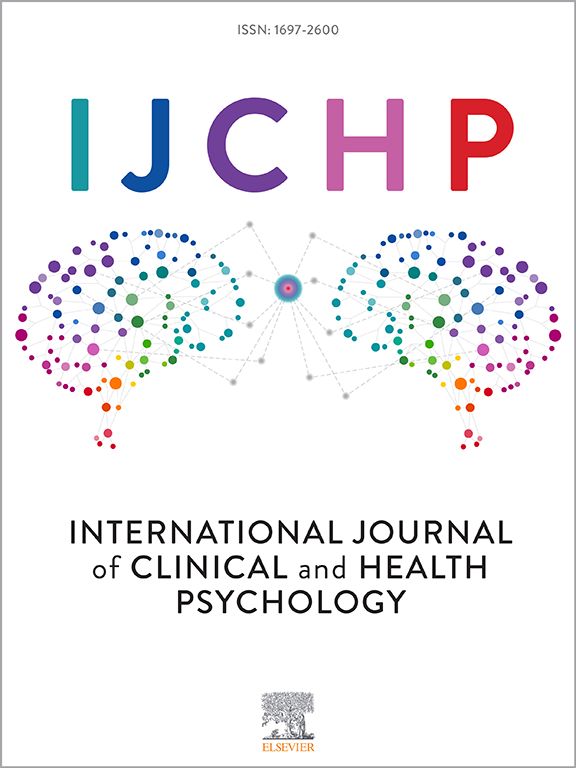
Edited by: Andre R Brunoni, Marie-Anne Vanderhasselt, Leigh Chavert
Last update: August 2022
More infoSelf-compassion has a consensual relevance for overall mental health, but its mechanisms remain unknown. Using intermittent theta burst stimulation (iTBS) and concurrent transcranial magnetic stimulation-electroencephalography (TMS-EEG), this study investigated the causal relationship of the dorsolateral prefrontal cortex (DLPFC) with self-compassion and explored the changes in neuroplasticity and neural dynamics.
MethodThirty-two healthy participants received iTBS or sham stimulation over the DLPFC, before and after which they were instructed to either use self-compassionate strategies or to be rejected in the context of social rejection and to report the level of self-compassion or negative affect. TMS-evoked potentials were evaluated as novel neuroplastic techniques with N45, P60, N100, and P180.
ResultsiTBS uniquely decreased P180 amplitude measured with TMS-EEG whereby sham stimulation had no effect on neuroplasticity. In line with neuroplasticity changes, iTBS enhanced a widespread gamma band power and coherence, which correlated consistently with increased engagement in self-compassion. Meanwhile, iTBS demonstrated opposite effects on theta activity dependent on the social contexts whereby self-compassion decreased and social rejection enhanced it respectively. This unique effect of iTBS on theta activity was also supplemented by the enhancement of theta band coherence following iTBS.
ConclusionsWe found a causal relationship between DLPFC and self-compassion. We also provide evidence to indicate widespread gamma activity and connectivity to correlate with self-compassion as well as the critical role of the DLPFC in modulating theta activity and negative emotions.
Self-compassion entails being warm and understanding toward oneself, recognizing that suffering is a part of shared human experience and taking a balanced view to negative emotions (Neff, 2003a; Neff, 2003b). A series of studies have demonstrated a positive relationship between self-compassion and emotional well-beings (Bates et al., 2021; Luo et al., 2018; Neff et al., 2020; Zhang et al., 2016). Self-compassion interventions have been explored as potential therapeutic tools in clinical settings (Friis et al., 2016; Kılıç et al., 2021; Kirby et al., 2017; Luo et al., 2021; Tian et al., 2020). It is therefore necessary to understand the neural underpinnings behind self-compassion in social contexts due to its consensual relevance for well-being and overall mental health (Neff, 2022).
A few studies have begun to explore the mechanistic evidence of self-compassion, in which the dorsolateral prefrontal cortex (DLPFC) seems to play a key role (Berry et al., 2020; Guan et al., 2021). For instance, trait self-compassion was associated with increased DLPFC response to self-criticism versus neutral contexts following a brief self-compassion training (Lutz et al., 2020). In another study, self-compassion correlated negatively with DLPFC activation while viewing sad versus neutral faces (Liu et al., 2020). A recent study using voxel-based morphometry analysis also revealed a negative correlation between dispositional self-compassion and gray matter volume in the left DLPFC (Guan et al., 2021). In addition, self-reassurance, a proxy for self-compassion, elicited greater activity in the DLPFC compared to self-criticism (Lutz et al., 2020). Overall, the role of the DLPFC in self-compassion and the exact influence remain to be established with more causal evidence. Besides, it is also important to investigate psychological and/or environmental factors, such as experimental protocols, in the implication of DLPFC in self-compassion.
Transcranial Magnetic Stimulation (TMS) is a safe and non-invasive form of brain stimulation which provides a window to investigate the causal role of brain activations in psychological processes. Theta-burst stimulation (TBS) is one of the most established repetitive TMS protocols and has the capacity to modulate neural excitability and connectivity (Che, Cash, et al., 2021; Che et al., 2021; Huang et al., 2005; Ni et al., 2014). Specifically, intermittent TBS (iTBS) is designed to increase cortical excitability with a burst mode (Huang et al., 2005). Moreover, TBS-induced neuroplastic changes in the prefrontal cortex can be measured with concurrent TMS and electroencephalography (TMS-EEG) by means of TMS evoked potentials (TEPs), such as N45, P60, N100, and P180 (Ahn & Fröhlich, 2021; Cash et al., 2017; Che et al., 2019). These components are believed to reflect the shifts in the inhibition-excitation balance in cortical circuits (Du et al., 2018a; Du et al., 2018b; Premoli et al., 2014) and have been demonstrated to be reproducible (Ozdemir et al., 2021b; Ozdemir et al., 2021a).
One previous report of widespread increase in gamma power (31-100 Hz) and phase-synchrony assessed expert Tibetan Buddhist meditators during states of loving-kindness and compassion (Lutz et al., 2004). As a core element of self-compassion, mindfulness is suggested to rely on gamma power activity supporting the process of attention (Berkovich-Ohana et al., 2012) and the integration of sensations (Lomas et al., 2015; Lutz et al., 2004). It is therefore important to evaluate the changes in gamma activity and connectivity that are modulated by TMS, which may help to reveal regional and network-based neural mechanisms underlying self-compassion. However, there is no direct evidence on the neural dynamics or neural networks associated with self-compassion. In addition, self-compassion has often been employed in the context of social distress and social rejection, in which frontal and central theta activity (4-8 Hz) have been correlated consistently with negative affect induced by these scenarios (Cristofori et al., 2013; van der Molen et al., 2017; van der Molen et al., 2018; van Noordt et al., 2015).
Using iTBS and concurrent TMS-EEG, this study was designed to investigate the causal relationship of the DLPFC with self-compassion. The changes in neuroplasticity and neural dynamics were also explored. A scenario imagination task was performed during which participants were instructed to either downregulate emotions using self-compassion strategies or to view social rejection scenarios. We hypothesized that iTBS would increase TMS-evoked potentials in the prefrontal regions as well as the engagement in self-compassion. It is also hypothesized that self-compassion would correlate with increased gamma power and coherence but with decreased theta power.
MethodsParticipantsA power analysis was conducted using G*Power (Faul et al., 2009) (F tests, ANOVA: repeated measures, within factors, α = 0.05, effect size = 0.3) based on a similar study (η2p = 0.08) (He et al., 2020). Results indicated that thirty-two participants would ensure 90% statistical power. Thirty-two healthy, right-handed (self-reported) adults (16 males: 21 ± 3.7 years, 16 females: 19.9 ± 1.2 years) were recruited in this study. Participants were screened to confirm no contraindications for TMS (Rossi et al., 2011). They were also free from anxiety or depression assessed by the Trait form of Spielberger's State-Trait Anxiety Inventory (STAI-T) and the Beck Depression Inventory Second Edition (BDI-II) respectively (Beck et al., 1996; Spielberger et al., 1983). Participants reported a mean score of 30.3 ± 10.0 in STAI-T and 6.9 ± 6.6 in BDI-II. All participants provided an informed consent prior to the experiment. This study was approved by the Ethics Committee in the Centre for Cognition and Brain Disorders of Hangzhou Normal University (2021031001) and was conducted in accordance with the Declaration of Helsinki.
Experimental design and procedureThis study was a two (time: Pre- and Post-stimulation) by two (TMS: iTBS and Sham) within-subject, randomized, and sham-controlled design. Participants visited the lab twice with an interval of 72 h or longer. The experimental procedures were the same for each session except for the TMS stimulation (i.e. iTBS or Sham), which was pseudorandomized across participants (Fig. 1). In each session, the scenario imagination task and concurrent TMS-EEG were performed before and after TMS stimulation.
Experimental design and procedure. (a) iTBS protocol. (b) Experimental procedure of each session. (c) Schematic diagram of the scenario imagination task. All four people in this image agreed to the presentation here. (d) Concurrent TMS-EEG recordings over the DLPFC with white noise to mask TMS click sound.
The scenario imagination task consisted of a ‘no-regulation’ block and a ‘self-compassion’ block which were randomized across participants as well as TMS conditions (Fig. 1c). Each block included 30 trials with 30 images being randomly presented across Pre- and Post-stimulation. Each trial started with a variable fixation (1-3 s), followed by the presentation of an image for 8 seconds, during which participants were asked to either think of themselves being the person (‘no-regulation’) or to downregulate the negative emotions using self-compassion strategies (‘self-compassion’, see below for instructions). They were then asked to rate the level of negative affect (‘no-regulation’) or self-compassion (‘self-compassion’) using a nine-point scale (1 - least, 9 - most).
The images used here were derived from an image database of social inclusion and exclusion in young Asian adults (He et al., 2018; Zhao et al., 2021; Zheng et al., 2021). A total of 60 images were randomly assigned to the two tasks (30 of each), with the valence and arousal of the two sets being matched (Supplementary Material, Method section 1). The images were presented in the center of the screen. Participants were seated in a slightly reclined chair with their faces positioned 0.5 m from the screen.
Participants were initially asked to write down three compassionate sentences to console a significant other who is being socially rejected by others. This compassionated self-talk protocol has been demonstrated to be able to induce one's own compassionate statements or styles (Luo et al., 2020; Petrocchi et al., 2017). Before the self-compassion task, participants were told that “In this section, please imagine yourself being the person being socially rejected. Try to comfort yourself using the compassionate sentences you just provided.” In the no-regulation task, participants were instructed as follows: “In this section, please think about yourself being the person highlighted in this image.”
EEG recordingsEEG was recorded using 32-channel electrodes (c rings) mounted on a cap (Braincap, Brain Products Amplifier, Munich, Germany) in a temperature-controlled, sound-attenuated, and electrically shielded room. Electrodes were referenced to FCz and grounded to AFz. Electrode impedances were kept below 10 kΩ throughout recording. The sampling rate was 1000 Hz for the scenario imagination task and 5000 Hz for TMS-EEG recordings respectively. Participants were listening to white noise through intra auricular earphones during TMS-EEG recordings. The sound level was adjusted to that individuals could barely hear TMS clicks at 110% resting motor threshold (RMT).
Transcranial magnetic stimulationA Magstim stimulator (Magstim Rapid2, United Kingdom) connected to a figure-eight coil was used for both iTBS and single-pulse TMS. Resting motor threshold (RMT) was obtained from the left motor cortex with coil positioned at 45° angle relative to the midline. RMT was measured as the minimum intensity to induce 5 out of 10 motor-evoked potentials (MEPs) > 0.05 mV recorded from the first dorsal interosseous muscles of the right hand. The DLPFC was located with F3 electrode with reference to the 10/20 system (Chung et al., 2019b; Fitzgerald et al., 2009). TMS-evoked potential (TEPs) were recorded using EEG during single-pulse TMS (65 pulses, 4s interval ± 10% jitter, 110% RMT), administered before and after iTBS. The iTBS protocol contained a burst of three pulses (50 Hz) repeated every 200 ms for 2 s with an 8 s break, totaling 600 pulses (Fig. 1a) and was applied at an intensity of 80% RMT. The coil was positioned at 90° relative to the midline with the handle pointing left (Fig. 1d). Sham stimulation was administrated using the same iTBS protocol, with the coil being flipped 90° so the right wing was against the F3 electrode (Chung et al., 2017).
EEG data analysisEEG data during the scenario imagination task were analyzed using EEGLAB (Delorme & Makeig, 2004) and custom scripts running on MATLAB platform (R2017b, the MathWork, USA). Malfunctioning channels were visually checked and removed. Data were bandpass filtered between 0.5 and 100 Hz and bandstop filtered between 48 and 52 Hz using zero-phase Butterworth filters which is able to compensate for the filter delay (Widmann et al., 2015). Continuous data were segmented based on the onset of the image (-1 to 9 s). The epochs were slightly longer than the window of interest (0-8 s) to assist time-frequency decomposition and baseline correction (percentage). Data were then re-referenced to the common average reference. Stereotyped artifacts such as eye movement, muscle activity, and eye blinks were identified by visual inspection of the temporal and spatial characteristic of the independent components following independent component analysis algorithm (FastICA). Removed channels were interpolated and epochs were inspected again.
Time-frequency analyses were performed using Hanning tapered “mtmconvol” method in FieldTrip toolbox (Oostenveld et al., 2011). Power was calculated in the range of 1-100 Hz in the time window of -1000 to 9000 ms and baseline corrected (-500 to -10 ms) for each trial before averaging trials in each condition for each subject.
EEG connectivity was calculated by computing the debiased weighted phase lag index (WPLI) based on the frequency representations obtained above. The WPLI is a measure of the phase coherence of two signals, based on the imaginary part of the cross-spectrum (Vinck et al., 2011). WPLI is suggested to be able to reduce sensitivity to additional, uncorrelated noise sources, such as volume conduction, as well as to increase statistical power to detect changes in phase-synchronization (Vinck et al., 2011). For each frequency, the WPLI was computed for each electrode pair. Connectivity values were then averaged to the frequency bands of interest, i.e. theta (4-8 Hz) (Cristofori et al., 2013) and gamma (31-100 Hz) (Lomas et al., 2015). Other frequency bands (delta: 1-3 Hz, alpha: 8-12 Hz, beta: 13-30 Hz) were further examined for exploratory purposes.
TMS-EEG data analysisTMS-EEG data were preprocessed as previously reported (Che et al., 2019; Rogasch et al., 2017). Data were epoched around the TMS pulses (-1000 to 2000 ms) and baseline corrected (-500 to 50 ms) (Hill et al., 2021). The large magnetic pulse was removed and interpolated (-5 to 20 ms). Data were downsampled to 1000 Hz and were visually inspected for bad channels and trials containing excessive muscle activities. In each session, epochs across pre- and post-stimulation were concatenated to avoid bias in component rejection of independent component analysis (Hill et al., 2018). A first round of FastICA was performed to remove large muscle artefacts and decay artefacts using semi-automated component classification algorithm (Rogasch et al., 2017; Rogasch et al., 2014). Data were then filtered using Butterworth filters (band-pass = 1-100 Hz; band-stop = 48-52 Hz), and epochs were manually inspected again. The second round of FastICA was performed to remove non-neural artefacts, such as eye blinks, eye movements, persistent muscle activity, and electrode noise. Interpolation was then applied for removed channels. Data were then re-referenced to the common average. Finally, data were segmented into initial blocks (pre- and post-stimulation) for each session.
StatisticsFor behavioral measures, repeated measures two-way ANOVAs (TMS: iTBS, Sham; time: Pre, Post) were conducted for self-compassion ratings and negative affect separately in SPSS (IBM Corp, Armonk, NY, version 22). Post-hoc pairwise comparisons were conducted using a Bonferroni correction (α ≤ 0.05).
For TEPs, non-parametric cluster-based permutation statistics were performed at a global level. This method is suggested to be able to control multiple comparisons across EEG channels and time (Maris & Oostenveld, 2007). Statistics were conducted on peaks of interest. Time windows for each peak were determined based on previous studies (N45 (40-55 ms), P60 (55-75ms), N100 (90-130ms) and P180 (160-210ms)) (Chung et al., 2017; Hill et al., 2017; Premoli et al., 2014b; Ye et al., 2022) as well as on the waveforms of our data (Fig. 2a). Comparisons were made between Pre- and Post-stimulation for each stimulation condition using paired T-tests. An observed statistics value was considered in the cluster permutation if it was below the threshold of 0.05 in at least two of the neighboring channels (Oostenveld et al., 2011). We performed 5000 iterations of trial randomization to generate the permutation distribution and a threshold of 0.025 (two-tailed) was used for evaluating the electrodes that exhibit a significant difference in power.
Baseline TEPs and changes following stimulation. (a) Butterfly plots of all electrodes with peaks of interest highlighted. The waveform in red line indicates the fronto-central area (FC1, FC2, Cz) for illustration purposes. Data were combined across the DLPFC- and Sham-stimulation. (b) Topographical voltage distribution for the peaks of interest. (c) iTBS reduced P180 amplitude from Pre- to Post-stimulation. Grand average TEP waveforms from the three fronto-central electrodes (FC1, FC2 and Fz) were illustrated. (d) Topoplot highlights the channels showing significant changes from Pre- to Post-stimulation. * indicates p < 0.025.
For time-frequency data, the same cluster-based permutation tests were applied to the time window of interest (0-8 s) in all channels. Comparisons were made between Pre- and Post-stimulation for each stimulation condition (iTBS or Sham) and scenario imagination condition (self-compassion task or no-regulation task). Statistical analysis was initially performed in theta (4-8 Hz) and gamma (31-100 Hz) band based on a prior hypothesis. Exploratory analyses were also conduct in other frequency bands (i.e. delta: 1-3 Hz, alpha: 8-12 Hz, beta: 13-30 Hz).
Statistical analyses of EEG connectivity were performed using the network-based statistic (NBS) toolbox (Zalesky et al., 2010). The NBS is a nonparametric technique which uses cluster analysis to perform null hypothesis testing across networks of values from pairs of potentially connected nodes (Zalesky et al., 2010). Paired T-test were conducted from Pre- to Post-stimulation for each stimulation condition (iTBS or Sham) in the self-compassion task and the no-regulation task separately. We generated 5000 permutations for statistical comparisons with a primary threshold set at p < 0.005, in order to guarantee only robust differences in connectivity between electrode pairs to be compared at cluster level (Bailey et al., 2018). The secondary threshold for electrode pairs was set at p < 0.025 (two-tailed) for family-wise corrected cluster testing.
Pearson's correlation analyses were conducted to evaluate brain-behavior relationships between changes in self-compassion ratings and iTBS-induced changes in EEG power, TEP amplitude as well as EEG connectivity across subjects.
Although we have carefully randomized TMS conditions across genders and participants, a supplementary analysis was conducted to examine the ordering effect. Specifically, analysis of covariance was performed with condition orders as the covariate (1 = “iTBS-Sham”; 2 = “Sham-iTBS”).
Linear regression models were further performed to examine the association between baseline negative affect and changes in P180, theta power and gamma power (see Results).
As our participants observed the same figures in the two TMS conditions, a two-way ANOVA (sequence: First session, Second session; time: Pre, Post) was conducted to evaluate the learning effect on self-compassion ratings and negative affect separately.
ResultsEffects of iTBS on TMS-evoked potentialsTime-domain signals were presented as butterfly plots as well as voltage distribution across the scalp (see Fig. 2a and Fig. 2b). Single-pulse TMS over the left DLPFC resulted in a series of negative and positive peaks including N45, P60, N100 and P180, in line with previous TMS-EEG studies assessing prefrontal plasticity (Che et al., 2019; Chung et al., 2018). Each peak showed scalp topography similar to previous studies (Rogasch et al., 2014), indicating the spreading of voltage distribution across time.
Cluster-based permutation statistics revealed that the amplitude of P180 was decreased by iTBS (P = 0.004) (Fig. 2c), surrounding the frontal areas where iTBS was delivered to (Fig. 2d). There were no changes in TEPs following Sham stimulation (Ps > 0.05). Individual data of P180 can be found in Supplementary Material Table S1. No significant relationship was found between changes of P180 amplitude and behavioral ratings.
Effects of iTBS on behavioral performanceFor self-compassion ratings, two-way ANOVA revealed a main effect of time (F1,31 = 5.13, P = 0.031, = 0.14) (Supplementary Material Fig. S1), suggesting that self-compassion was increased (PBonf = 0.03) from Pre- (Mean = 6.55) to Post-stimulation (Mean = 6.74) in both the iTBS and Sham stimulation. Further analysis indicated that this time effect on self-compassion was driven by the changes in the iTBS condition (∆iTBS = 0.34, ∆Sham = 0.04, Fig. 3a).
Self-compassion changes as well as gamma power and coherence during the self-compassion task. (a) Self-compassion by time and stimulation. The time effect on self-compassion was driven by the changes in the iTBS condition (∆iTBS = 0.34, ∆Sham = 0.04). * denotes p = 0.024. (b) EEG power indicated increased gamma power at 5.9 s in the iTBS compared to the Sham stimulation (P corrected = 0.015). The topography of this power increase was located at frontal and centroparietal regions (significant at Fz, FC2, F4, P3, P4, Pz, CP2, CP6 and O1). (c) Increased gamma power positively correlated with self-compassion changes from Pre- to Post-stimulation across stimulations (r = 0.25, df = 62, p = 0.044). Blue dots mean the data of each participants in the iTBS condition while red dots represent individuals’ data in the Sham condition. (d) iTBS increased frontal and central gamma connectivity (F4-C3) (P corrected = 0.013, 5-7.5 s). Large dots highlight the significant electrodes, and the color of the lines indicates T statistics. (e) Increased gamma coherence correlated positively with engagement in self-compassion from Pre- to Post-iTBS (r = 0.36, df = 30, p = 0.046). Blue dots mean the data of each participants in the iTBS condition.
For negative affect, two-way ANOVA revealed a trend Condition × Time interaction effect (F1,31 = 3.94, P = 0.056, ηp2 = 0.11) (Supplementary Material Fig. S2). Pairwise comparisons found no difference between Pre- and Post-stimulation either in the iTBS (PBonf = 0.38) or Sham condition (PBonf = 0.10).
Effects of iTBS on EEG powersIn the self-compassion task, cluster-based permutation tests on power changes (Post - Pre) indicated increased gamma power at 5.9 s in the iTBS compared to the Sham stimulation (P corrected = 0.015) (Fig. 3b). The topography of this power increase was located at the frontal and centroparietal regions (significant at Fz, FC2, F4, P3, P4, Pz, CP2, CP6 and O1). Moreover, changes in gamma power correlated with increased self-compassion (r = 0.25, df = 62, p = 0.044) in the pooled dataset of the two stimulation conditions (Fig. 3c).
Further analyses revealed a significant decrease in theta power during 7.5-7.8 s from Pre- to Post-iTBS (P corrected = 0.022) (Fig. 4a). The topography of this power change was mainly distributed in the right frontal areas (significant at Fz, FC2, and F4). Moreover, the decrease in P180 amplitude correlated with decreased theta power induced by iTBS (r = 0.41, df = 30, p = 0.021) (Fig. 4b). No difference was found in any other frequency bands.
iTBS-induced changes in theta band power and connectivity dependent on the social contexts. In the self-compassion task: (a) time frequency analysis indicated decreased frontal theta power from Pre- to Post-iTBS (P corrected = 0.022, significant at Fz, FC2 and F4 during 7.5 s to 7.8 s). (b) Decreased P180 amplitude correlated with decreased theta power from Pre- to Post-iTBS (r = 0.41, df = 30, p = 0.021). Blue dots represent the data of each participants in the iTBS condition. (c) iTBS increased theta band coherence in the fronto-frontal and fronto-occipital regions anchoring in the right prefrontal cortex (F8) (P corrected = 0.006, 7.1-8 s). Large dots highlight the significant electrodes and the color of the lines indicates T statistics. In the no-regulation task: (d) time frequency analysis indicated increased theta power at 7.4 s from Pre- to Post-iTBS (P corrected = 0.018), distributing over the centroparietal regions (significant at F3, FC1, F7, C3, Cz, P4, P8, T7, T8, CP1, CP2, CP6, CPZ, TP9, and TP10). (e) Increased theta power correlated with more negative affect from Pre- to Post-iTBS (r = 0.37, df = 30, p = 0.039). Blue dots represent individuals’ data in the Sham condition. (f) iTBS increased theta band coherence between the prefrontal and central cortices (P corrected = 0.016, 7-8 s). Large dots highlight the significant electrodes and the color of the lines indicates T statistics.
In terms of no-regulation task, we found increased theta powers at 7.4 s from Pre- to Post-iTBS (P corrected = 0.018), which mainly distributed in the centroparietal regions (significant at F3, FC1, F7, C3, Cz, P4, P8, T7, T8, CP1, CP2, CP6, CPZ, TP9, and TP10) (Fig. 4d). Increased theta power correlated with more negative affect from Pre- to Post-iTBS (r = 0.37, df = 30, p = 0.039) (Fig. 4e). No difference was found in any other frequency bands.
Effects of iTBS on EEG connectivityIn the self-compassion task, iTBS also increased gamma band coherence between the prefrontal and central cortices (F4-C3) (P corrected = 0.013, 5-7.5 s) (Fig. 3d). In the same task, iTBS resulted in increased fronto-frontal and fronto-occipital connectivity in the theta band anchoring in the right prefrontal cortex (P corrected = 0.006, 7.1-8 s) (Fig. 4c). Moreover, increased gamma band coherence correlated with increased self-compassion changes following iTBS (r = 0.36, df = 30, p = 0.046) (Fig. 3e).
In the no-regulation task, iTBS increased frontocentral connectivity in the theta band (P corrected = 0.016, 7-8 s) (Fig. 4f). No other significant clusters were observed by iTBS and no significant clusters were observed in the Sham stimulation.
Supplementary analysisIn the exploration of ordering effect, the main effect of time remained the same (F1,30 = 4.59, P = 0.04, ηp2 = 0.13), with no significant effect of the testing order (Ps > 0.05).
Baseline negative affect was able to predict theta power changes in no-regulation task (R = 0.35, B = -0.86, P = 0.047). No significant result was found on P180 or other EEG power changes.
For self-compassion ratings, two-way ANOVA revealed no main effect of session sequence or any interaction effect (Ps > 0.05). For negative affect ratings, no effect was observed (Ps > 0.05).
DiscussionUsing iTBS, this study was designed to investigate the influence of the DLPFC on self-compassion as well as the changes in neural dynamics and brain networks. In terms of frontal plasticity, iTBS uniquely reduced P180 amplitude measured with TMS-EEG whereby sham stimulation had no effect. Moreover, iTBS increased a widespread gamma band power and coherence that correlated with the engagement in self-compassion. Meanwhile, iTBS demonstrated distinct modulating effects on frontal theta power dependent on the social contexts whereby self-compassion scenarios decreased and social rejection enhanced it respectively. This unique effect of iTBS on theta activity was also demonstrated by the enhancement of theta band coherence following stimulation.
In the current study, iTBS over the DLPFC uniquely decreased P180 amplitude in the frontal cortices whereas no such effect was observed in the sham stimulation (Fig. 2c). This is consistent with previous studies which demonstrated similar P180 changes in the DLPFC using rTMS (Chung et al., 2019a; Chung et al., 2019b; Zrenner et al., 2020). Although the origins and functional significance of P180 have not been fully understood, P180 is suggested to be correlated with axonal excitability as its amplitude is particularly reactive to excitability-lowering drugs such as classic voltage-gated sodium channel (VGSC) blockers, but not to GABAergic drugs (Darmani et al., 2019a; Darmani & Ziemann, 2019b; Premoli et al., 2017). Our finding of P180 change therefore provides further evidence to demonstrate the effects of iTBS in modulating prefrontal excitability. Although deceased P180 amplitude replicates the literature (Chung et al., 2019a; Zrenner et al., 2020), we did not find a direct relationship between changes in P180 amplitude and behavioral measurements. There was a significant positive correlation between changes in P180 and theta power in the self-compassion task. It is possible that neuroplastic changes induced by TMS have a more indirect modulation on behavioral measurements through unique neural responses to the task demand.
In consistent with the neuroplastic changes, self-reported self-compassion was improved by both the iTBS and sham stimulation (Supplementary Material Fig. S1), whereby this effect was more prominent in the iTBS condition (Fig. 3a). Previous studies have observed a correlation between DLPFC activation and self-reported self-compassion (Berry et al., 2020; Lutz et al., 2020). Our data therefore provide evidence to indicate a more causal relationship of the DLPFC in self-compassion when dealing with social distress. Although our data was not able to exclude the possibility of a practice effect, the improvement of self-compassion was more prominent in the iTBS condition and related to unique changes in neural dynamics and connectivity (see discussions below). These findings further corroborate the role of the DLPFC in modulating self-compassion.
Interestingly, iTBS increased a widespread gamma activity distributing over the frontal and centroparietal regions during self-compassion (Fig. 3b). An early study suggested prefrontal and midline gamma powers to be associated with the process of self-referencing and attention in mindfulness (Berkovich-Ohana et al., 2012). Indeed, gamma powers are widely suggested to integrate distributed neural processes into highly ordered cognitive functions (Başar et al., 2001; Keil et al., 2001). It is noted that the participants were instructed to think of themselves being socially rejected and to comfort themselves with self-compassion strategies in the current study. Increased gamma activity in our data thus fits nicely to the integrating role and potentially provides oscillatory correlates for self-compassion processes. This argument is further supported by our data in which there was a positive relationship between changes in gamma power and self-compassion following stimulation (Fig. 3c).
We also provided strong evidence of increased gamma band synchronicity during self-compassion following iTBS (Fig. 3d). This pattern of coherence change fits nicely to the observed gamma powers both in terms of frequency and spatial distribution (Fig. 3d). Rhythmic synchronization of neural discharges in the gamma range is believed to contribute to the integration of sensations and experiences into a coherent state of moment-to-moment awareness (Lomas et al., 2015; Singer, 1993; Tallon-Baudry & Bertrand, 1999). It is proposed that selective attention induces stronger and higher-frequency gamma band synchronization which renders effective and precise communication (Fries, 2015). Increased gamma band coherence between the frontal and central cortices may serve to employ compassionate strategies in the management of negative emotions aroused by social distress. This was further supported by the positive association between increased gamma band coherence and the engagement in self-compassion (Fig. 3e). Overall, we provide multiple lines of evidence that iTBS over DLPFC is able to modulate the engagement in self-compassion and that gamma band power and coherence to be potential mechanisms of self-compassion.
There is evidence to indicate the involvement of gamma power in compassion (Lutz et al., 2004). A significant positive relationship was found between arousal scores and gamma power in individuals with high trait of empathy during the presentation of compassion clips (Maffei et al., 2019). Our results further provide strong evidence on the link between self-compassion and gamma power as well as coherence. It remains an open question whether compassion for others and self-compassion are in fact part of the same overarching construct (Anālayo & Dhammadinnā, 2021; Lee et al., 2021; MacBeth & Gumley, 2012). While some studies indicate compassion to be synonymous with self-compassion in mental health (MacBeth & Gumley, 2012), others found no relationship between self-compassion and compassion for others (López et al., 2018). A recent review failed to identify the DLPFC as neuro activation of compassion (Kim et al., 2020). However, the DLPFC seems to be a critical region in self-compassion. This issue might be further understood in the view of brain activity, especially of gamma activity, in future studies (Stevens & Woodruff, 2018).
Our data also revealed decreased frontal theta power in the late stage of a self-compassion episode (Fig. 4a). Event-related theta power has been associated with a variety of cognitive processes such as behavioral adaptation (Cavanagh et al., 2010; Cavanagh & Shackman, 2015), attention (Clayton et al., 2015), working memory (Kahana et al., 2001; Khader et al., 2010; Sauseng et al., 2010) and emotion regulation (Aftanas et al., 2001; Aftanas et al., 2003; Aftanas et al., 2004). Using social rejection paradigms similar to ours, some studies demonstrated increased theta activity to be associated with negative affect induced by social rejection (Tang et al., 2019; van Noordt et al., 2015). The spatial (i.e. frontal regions) and temporal (i.e. late stage) patterns of decreased theta in our data align nicely to literature demonstrating its role in negative emotions (Bekkedal et al., 2011; Uusberg et al., 2014). Moreover, decreased theta power correlated with the suppression of P180 amplitude in the exact same regions following iTBS (Fig. 4b). Overall, our data provide evidence on the modulation of theta power potentially associated with the effects of self-compassion on negative emotions. It is noted that increased theta power may be preferentially underlying focused-attention meditation (Brandmeyer & Delorme, 2018, 2020; DeLosAngeles et al., 2016; Lee et al., 2018; Mitchell et al., 2008; Yoshida et al., 2020) In this study, self-compassion may display unique neural activity which is different from focused attention meditation.
In addition to theta power, our data also demonstrated increased theta band coherence between frontal and occipital regions during self-compassion following iTBS (Fig. 4c). This finding further corroborates self-compassion in the modulation of frontal theta power associated with negative affect. Moreover, our data highlighted right prefrontal cortex in orchestrating information changes between the prefrontal and occipital regions. This pattern of coherence is consistent with the finding whereby the right prefrontal cortex is critical for the management of negative emotions in social exclusion (He et al., 2018; He et al., 2020). It is also interesting to find that occipital regions are involved in theta band coherence, which may be associated with the presentation of visual stimuli and/or upcoming ratings in this study. In the self-compassion task, visual presentation of the image initially leads to the processing of the content. Emotion regulation might then follow with self-compassion strategies. It is therefore interesting to find that the effects on gamma power and coherence associated with self-compassion happened around 5-7.5s in the late stage of the protocol. The effects on theta power and coherence at 7.1-8s might be correlated with decreased negative affect following self-compassion. Moreover, increased theta power in the no-regulation task at 7.4s corelated with increased negative affect further corroborates this argument.
As an active control, participants were instructed to think of themselves being socially rejected, which correlated with increased theta power in the late phase of the scenario following iTBS stimulation (Fig. 4d). Theta power has been associated with a variety of cognitive and emotional functions (McNaughton et al., 2007). Midfrontal theta is believed to reflect the need for control especially in the situations that elicit anxiety (Cavanagh & Frank, 2014; Cavanagh & Shackman, 2015). In fact, frontal-midline theta is increased by anxiolytic drug action and personality-related reductions in anxiety (Mitchell et al., 2008). Moreover, the occurrence of theta activity during negative affect might be underlying adaptive behavioral adjustments (Cavanagh & Shackman, 2015). In this study, participants reported negative affect which is not a specific emotion. However, as discussed earlier, previous studies have demonstrated increased theta activity in response to social rejection and associated negative emotions (Cristofori et al., 2013; van der Molen et al., 2017; Zhang et al., 2021). Built on this, we here further demonstrated a critical role of the DLPFC in the processing of social rejection and negative emotions whereby iTBS enhanced frontocentral theta activity and induced a covarying change in negative affect in the contexts of social rejection (Fig. 4e). This argument is further supplemented by enhanced theta band connectivity following iTBS (Fig. 4f). Moreover, the spatial distribution of theta connectivity is consistent with the changes in theta power spanning from the left prefrontal to right central cortices. Overall, our data extend previous findings by demonstrating that iTBS is able to modulate theta band power and coherence associated with negative emotions.
Regression analysis showed that baseline negative affect is able to predict theta power changes in no-regulation task. This finding further confirms the association between negative affect and theta power activity. Our supplementary analysis revealed no significant effect of session sequence which is able to exclude the learning effect. It is possible that the time interval between two sessions is long enough to avoid carryover effect.
There were some limitations in the study. Self-compassion is a complex cognitive process potentially involving multiple brain regions such as the medial prefrontal cortex and ventrolateral prefrontal cortex (Kim et al., 2020; Longe et al., 2010). These areas could be further explored in self-compassion using brain stimulation technologies. The self-compassion and imagining paradigm used here have been proved to be able to induce self-compassion and negative affect respectively (Luo et al., 2020; Ochsner et al., 2004; Zhao et al., 2021). Future studies may wish to validate our findings with other paradigms such as the Cyberball and online speed dating (Williams & Jarvis, 2006; Zhang et al., 2021).
Our results may have clinical implications. TMS could be combined with psychological interventions in order to improve clinical outcomes. A preliminary study reported success for combining TMS with exposure therapy in the treatment of posttraumatic stress disorder (Fryml et al., 2019). Our results demonstrated the influence of iTBS on self-compassion and gamma power and connectivity, which may have implications for optimizing the efficacy of self-compassion interventions in clinical settings. Moreover, some studies indicated impaired functioning of the DLPFC in the downregulation of negative affect in clinical settings (Glausier & Lewis, 2018; Phillips et al., 2008; Rive et al., 2013; Zilverstand et al., 2017). Our findings revealed the influence of the DLPFC on self-compassion as well as the neural dynamics and coherence associated with negative affect, thus providing evidence for targeting the DLPFC for the treatment of emotional disorders.
In conclusion, this study revealed the causal influence of the DLPFC in modulating the engagement in self-compassion. We found a widespread gamma band power and coherence to be associated with self-compassion. In addition, DLPFC had a distinct modulating impact on theta activity dependent on the social contexts of self-compassion or social rejection.
HL is supported by the Science and Technology Innovation 2030 Major Project (2021ZD0200520), the Guangdong Key Project (2018B030335001) and the Ministry of Education's Philosophy and Social Science Research Major Project (21JZD063). XC is supported by the National Natural Science Foundation of China (4045F41120040), and Hangzhou Municipal Health Commission (2021WJCY130). This work was also supported by the Key Research and Development Program of Zhejiang Province (2022C03038).