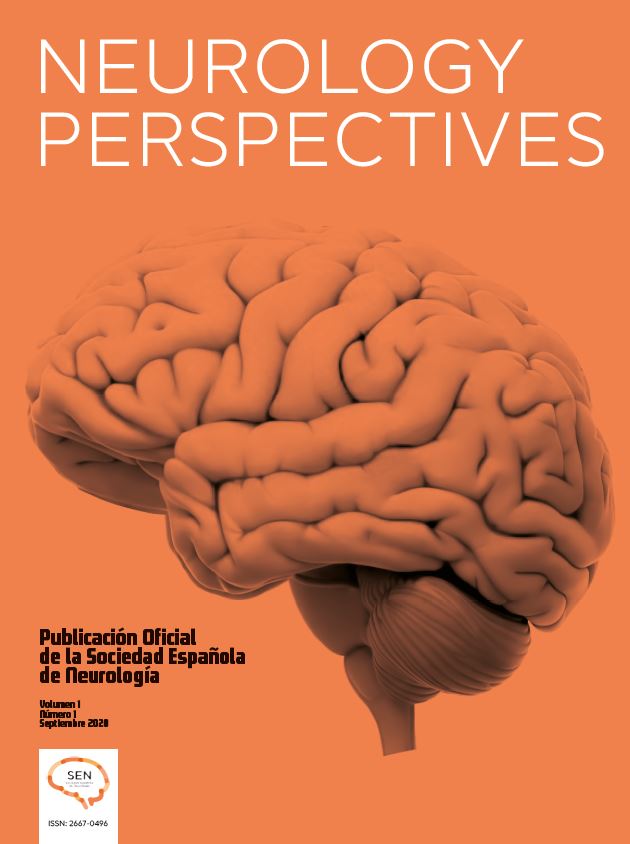
Biomaterial and stem cell -based treatment strategies for diseases of the central nervous system
Más datosNeonatal hypoxic-ischaemic brain injury is one of the main known causes of cerebral palsy, the most frequent and severe form of motor disability in childhood. Hypoxia-ischaemia induces inflammation, oxidative stress, and excitotoxicity, which ultimately compromises neuronal and oligodendroglial survival and viability. No effective treatment is currently available for the condition, and the effect of therapeutic hypothermia is very limited. Recent studies have proposed stem cell therapy as a potential strategy for preventing the condition.
Material and methodsMost preclinical studies have used Rice-Vannucci rodent models.
ResultsOur results showed that, depending on the timing of treatment onset, stem cells had either neuroprotective or neurorestorative effects, and ultimately recovered motor function. Although the dosage and route of administration may vary, this therapy reduced the extent of cell damage and death by attenuating the release of cytotoxic mediators. Clinical and preclinical trials have confirmed that umbilical cord stem cells and mesenchymal stem cells present sufficient efficacy and safety to be considered a potential treatment option.
ConclusionWe present our findings on stem cell therapy for cerebral palsy secondary to neonatal hypoxic-ischaemic brain injury.
El daño cerebral hipóxico isquémico (HI) neonatal es una de las principales causas conocidas de Parálisis Cerebral infantil (PCI), la discapacidad motora más grave y frecuente durante la infancia. Como consecuencia, se produce un aumento de la inflamación, el estrés oxidativo y la excitotoxicidad que, en última instancia, comprometerán la supervivencia y viabilidad neuronal y oligodendroglial. Hoy en día no existe una terapia eficaz que logre reducir este daño más allá de la hipotermia terapéutica, cuyo efecto es muy limitado. En este sentido, recientes estudios han propuesto la terapia con células madre como una potencial estrategia a la hora de prevenir este trastorno.
Material y MétodosLa mayor parte de los ensayos preclínicos se han desarrollado en roedores, siguiendo el modelo de Rice Vannucci.
ResultadosLos resultados demostraron que, dependiendo del momento de inicio del tratamiento, las células madre ejercieron un efecto neuroprotector y neurorreparador que última instancia recuperó la funcionalidad motora. A pesar de que la vía y la dosis administrada puede variar esta terapia ha logrado reducir el volumen de daño y la muerte celular atenuando los mediadores citotóxicos liberados. A su vez, los ensayos clínicos y preclínicos han confirmado que especialmente las células derivadas de cordón umbilical y las células mesenquimales presentan unos estándares de eficacia y seguridad que permiten postularlas como una posible terapia futura.
ConclusiónEste trabajo recoge los principales datos obtenidos sobre el tratamiento con células madre en la PCI secundaria al daño HI.
The neonatal period spans from birth to the fourth week of life.1–4 This stage, considered the most vulnerable stage of infancy, is when the risk of developing cerebral palsy (CP) is highest.1–4 CP is the most severe and most frequent form of motor disability in childhood. However, recent studies have shown that these patients frequently present other comorbidities, including hyperalgesia, cognitive impairment, and epilepsy.1–4 One of the main known causes of CP is diffuse or focal hypoxia-ischaemia.1–6
Overall, nearly 2 million infants with hypoxic-ischaemic brain injury die or develop long-lasting disabling sequelae, including motor and cognitive deficits, each year; the condition greatly affects the lives of these children and their families and has a major socioeconomic impact.4,7,8 Although hypoxia-ischaemia is frequent, the incidence and severity of its sequelae have not decreased over the years.4 Its complex pathophysiology has hindered the development of treatments targeting all the pathogenic mechanisms simultaneously.1–4 Furthermore, the condition is usually silent; by the time it is diagnosed, it is frequently too late to act.1–4 Lastly, the currently available treatments not only have limited effectiveness and a narrow therapeutic window, but may also cause severe adverse effects that may impair the child’s development.1–4
Hypoxic-ischaemic brain injury is a process caused by reduced cerebral blood flow, which leads to insufficient supply of oxygen and glucose to the brain. This causes primary energy failure and increased anaerobic metabolism, which results in increased lactate levels (increased lactic acidosis) and decreased ATP concentration (Fig. 1). Blood flow, pH, and metabolite and ATP levels subsequently return to baseline levels.1–4 However, a second energy failure occurs some time later as a consequence of mitochondrial dysfunction and increased oxidative stress, inflammation, and excitotoxicity; these pathophysiological events may last several hours, and even days (Fig. 1). Brain damage is caused by astrocytes and microglia. These cells release cytotoxic mediators that alter the integrity and survival of oligodendrocytes,2,4,6,9–12 which form myelin; myelin damage is a pathological hallmark of hypoxia-ischaemia and is responsible, at least partially, for the sequelae of CP.2,4,6,9–11 It is therefore essential to develop treatments able to reduce cytotoxic damage and promote oligodendrocyte survival, with the ultimate goal of preserving white matter integrity (Fig. 1).
Pathophysiology of hypoxic-ischaemic brain injury. Neonatal hypoxic-ischaemic brain injury is characterised by axonal dysfunction resulting from damage to oligodendroglial lineage cells and ultimately to myelin. Damage is mainly caused by energy failure and the release of such cytotoxic mediators as reactive oxygen species and proinflammatory molecules through the action of astrocytes (red) and microglia (yellow). Created with BioRender.
Besides therapeutic hypothermia, no other treatment is currently able to reduce the sequelae of hypoxia-ischaemia.1–6 Even therapeutic hypothermia has numerous disadvantages given that it only has positive effects when administered within 6 hours of the event, and only in mild cases; its therapeutic potential is therefore limited.4 Other treatments with demonstrated efficacy in preclinical trials, such as erythropoietin and melatonin, have not been demonstrated to be effective in clinical practice.4
Stem cell therapyIn this context, stem cell therapy has been proposed as a potential option for preventing the sequelae of neonatal hypoxia-ischaemia, as it may be used to modulate the underlying pathophysiological events and/or promote cell survival.
The therapeutic use of stem cells is somewhat controversial in some cases.13–16 Such issues as the most appropriate dose, route of administration, and timing of treatment onset are still unclear.17–19 In fact, recent studies suggest that stem cells have a neuroprotective effect when treatment is started within 24 hours of hypoxic-ischaemic injury, acting against the pathophysiological processes described above.17,20,21 However, when treatment is started more than 24 hours after the event, stem cells exert a neurorestorative effect, promoting neurogenesis, vasculogenesis, and brain tissue formation (Fig. 2).17,20,21
Therapeutic effects of stem cell therapy. Stem cell therapy administered within 24 hours of brain injury has a protective effect as it reduces astrogliosis (1) and microgliosis (2), which in turn reduces the levels of proinflammatory cytokines (3). It also prevents the increase in the levels of reactive oxygen species (4) and cell death by inhibiting caspase release (5). Stem cell therapy administered within 24 hours of the injury promotes neurorepair through cell proliferation and differentiation (6), neurogenesis (7), angiogenesis (8), and the release of trophic and anti-inflammatory factors (9). Created with BioRender.
Given that the efficacy and the most appropriate route of administration and timing of treatment onset vary depending on the cell type used,17–19 we summarise the main preclinical and clinical trials conducted to date into the prevention of CP secondary to neonatal hypoxic-ischaemic brain injury by cell type.
Umbilical cord blood cellsFor many years, umbilical cord solid tissue was treated as medical waste.19,22,23 However, umbilical cord blood has recently been identified as a valuable source of stem cells and haematopoietic precursor cells.19,22,23 Haematopoietic stem cells, characterised by presence of the CD34 marker, are heterogeneous cells from different lineages and at different stages of maturation that present a high capacity for self-renewal.19,22,23 Furthermore, these cells, unlike other types of stem cells, are easily obtained through non-invasive means, are easy to store, and their donation poses no risk to the donor and is highly unlikely to transmit clinically relevant infections to the recipient.19,22,23 Furthermore, compared to adult peripheral blood, umbilical cord blood has been found to decrease the immune response to alloantigens and rarely causes graft-versus-host disease.19,22,23 However, umbilical cord blood collection should be optimised to increase the amount of cells obtained.19,22,23
Most preclinical studies into hypoxic-ischaemic brain injury with umbilical cord blood stem cells have used rats (Table 1).17,24–29 These studies use the Rice-Vannucci model, in which animals undergo unilateral common carotid artery ligation and are subsequently exposed to a hypoxic environment.24,30 In exceptional cases, these studies have also been conducted in sheep and rabbits.17,31,32 Stem cells are generally administered intraperitoneally or intravenously,17,24–28,33 at a dose ranging from 106 to 107 cells, with no significant differences observed between doses.17,24–29,33 When treatment was started within 24 hours of hypoxic-ischaemic injury, it was shown to have a neuroprotective effect as it reduced the levels of inflammation and oxidative stress.17,25,31,32,34 However, when treatment was started beyond 24 hours after injury, animals displayed fibroblast proliferation, neuronal maturation, synaptic enhancement, increased angiogenesis, and restored blood flow and blood–brain barrier permeability.17,24,26–29 Most trials were conducted at 24 hours, when a synergistic effect occurs and stem cells present both a neuroprotective effect, reducing the levels of proinflammatory cytokines, and a neurorestorative effect, promoting the maturation of neurons and neuronal synapses.17,24,26–29 This resulted in a decrease in mortality and lesion volume, with animals recovering motor and cognitive function.17,24–29
Main preclinical trials of stem cell therapy for neonatal hypoxic-ischaemic brain injury.
Cell type (species) | Species | Route of administration | No. cells | Treatment onset | Model |
---|---|---|---|---|---|
UCB (human, mouse) | Rat, mouse, rabbit, sheep | IP, IT, IVT, IA, IN, IV | 1.5 × 104 - 108 | 3 h–3 w post-injury | RV, UI, UCO |
UCT MSCs | Rat, mouse | IP, IVT, IN, IV | 5 x 103 –5 x 106 | 1 h–5 d post-injury | RV, IVH, PWMD |
UCB MSCs | Rat | IVT | 105 | 6 h–3 d post-injury | RV, MCAO |
PT MSCs | Rat | IVT | 5 × 104–106 | 2 d post-injury | RV |
EPCs/ECFCs | Rat, mouse | IP | 105–5 × 105 | 1–2 d post-injury | RV |
NPCs | Mouse | IC | 2.5 × 105 | 2 d post-injury | RV |
NSCs (human, rat, mouse) | Rat, mouse | IC, IVT | 105–9.6 × 105 | 1–10 d post-injury | RV |
OPCs derived from MSCs (mouse) | Rat | IVT | 2.5 × 105 | 2 h post-injury | RV |
BM MSCs (human, mouse) | Rat, mouse, lamb fetus | IVT, IN, IV, SC | 0.8 × 105–6 × 106 | 1 h–17 d post-injury | RV, MCAO, UCO |
BM: bone marrow; d: days; ECFC: endothelial colony forming cells; EPC: endothelial progenitor cell; h: hours; IA: intraarterial; IC: intracerebral; IN: intranasal; IP: intraperitoneal; IT: intrathecal; IV: intravenous; IVT: intraventricular; IVH: intraventricular haemorrhage; MCAO: middle cerebral artery occlusion; MSC: mesencephalic stem cell; NPC: neural progenitor cell; NSC: neural stem cell; OPC: oligodendrocyte precursor cell; PT: placental tissue; PWMD: periventricular white matter damage; RV: Rice-Vannucci model; SC: subcutaneous; UCB: umbilical cord blood; UCO: umbilical cord occlusion; UCT: umbilical cord tissue; UI: uterine ischaemia; w: weeks.
The clinical trials conducted to date have shown that infusion of autologous umbilical cord blood stem cells is safe, due to the lack of adverse reactions.23,34 Furthermore, most treatments have been administered in several doses, starting 24 hours after hypoxic-ischaemic injury (Table 2). This treatment has also been found to be safe when combined with hypothermia; however, no in vivo studies have demonstrated whether the efficacy of both treatments improves when they are administered in combination.17–19,23,34
Main clinical trials of stem cell therapy for neonatal hypoxic-ischaemic brain injury.
Cell type | Trial no. | Treatment onset (hypothermia) | Administration route | Trial objective |
---|---|---|---|---|
Umbilical cord blood–derived stem cells | NCT02455830* | 3 doses within 72 hours (yes) | IV | To study the viability and safety of umbilical cord blood stem cell therapy in neonates with hypoxic-ischaemic brain injury |
NCT02256618 | 3 doses within 72 hours | |||
NCT02881970 | Within 3 days (yes) | |||
NCT00593242 | 4 doses within 14 days (yes) | |||
*: To measure the levels of inflammatory cytokines and trophic factors associated with perinatal brain injury and its repair | ||||
NCT03352310 | One dose within 48 hours of birth | |||
NCT02551003 | 3 doses within 72 hours (yes) | |||
NCT02612155 | 2 doses within 48 hours (yes) | |||
CL2020 (from adipose tissue) | NCT04261335 | 5-14 days after birth (yes) | IV | To assess the efficacy and safety of CL2020 cells for the treatment of hypoxic-ischaemic brain injury and for infant development |
Neural progenitor cells and paracrine factors | NCT02854579 | 3 doses of paracrine factors at 12, 24, and 48 hours after birth, and 3 doses of neural progenitor cells at 48-72 hours, 5 days, and 10 days after birth (yes) | IT | To evaluate the efficacy and safety of allogenic neural progenitor cells and paracrine factors of human mesenchymal stem cells for the treatment of hypoxic-ischaemic brain injury |
Human umbilical cord tissue–derived mesenchymal stem cells | NCT03635450 | 1st dose within 48 hours of birth | IV | To determine the safety of single and repeated intravenous doses of human umbilical cord tissue–derived mesenchymal stem cells for hypoxic-ischaemic brain injury |
2nd dose at 2 months of birth |
In conclusion, clinical and preclinical trials have shown the efficacy and safety of stem cell therapy, which constitutes a promising strategy for preventing CP, as it reduces the pathophysiological processes and promotes neuronal maturation.
Mesenchymal stem cellsAs is the case with haematopoietic stem cells, mesenchymal stem cells (MSC) are undifferentiated multipotent cells that can subsequently differentiate into different lineages, including osteogenic, adipogenic, chondrogenic, and haematopoietic lineage cells.17–19,22,23 However, in the context of hypoxic-ischaemic brain injury, this cell type has also been observed to differentiate into neurons.19 The placenta, umbilical cord tissue or blood, adipose tissue, and amniotic fluid have been identified as rich sources of MSCs.17–19,22,23 Furthermore, the risk of thrombus formation or graft-versus-host disease is low, given that the cells are derived from neonatal tissue.19
Treatment with these cells has been found to recover motor function in diseases with similar pathophysiological mechanisms (Table 1).19 Below, we review the preclinical trials conducted to date on animal models of hypoxic-ischaemic brain injury treated with mesenchymal stem cells of different origin.
Mesenchymal stem cells from umbilical cord tissueThe umbilical cord (cord lining, the perivascular region, Wharton’s jelly, etc) is one of the main sources of MSCs.19 These cells present a high proliferation rate, express chemokines and angiogenic growth factors, secrete trophic factors, and present marked immunomodulatory activity.19,35–37
Many preclinical trials with umbilical cord tissue–derived MSCs have used rats with hypoxic-ischaemic brain injury (Rice-Vannucci model).19,35,36,38–41 Rats were administered 105 to 106 cells19,35,36,38–41; however, unlike in the case of trials of umbilical cord blood cells, these cells were most frequently administered via the intraventricular route.19,35,37–40 Furthermore, treatment was started between 1 and 5 days after injury, and cells were administered in a single dose.19,35,36,38–41 This treatment was found to recover motor and cognitive function, reduce brain damage, and decrease the levels of apoptotic markers due to the decrease in microgliosis and astrogliosis, and consequently in inflammation.19,35,36,38–41 Better results were also observed with earlier treatment onset, as this increased the release of trophic and angiogenic factors.19,35–37
To date, umbilical cord tissue stem cells are the only MSCs that have been used in clinical trials.19,42 A phase 1 study conducted at Duke University (NCT03635450) showed that intravenous administration of these cells was safe for children with hypoxic-ischaemic brain injury19,42 when administered in 1 or 2 doses and at 48 hours or 2 months after injury, respectively (Table 2).
Mesenchymal stem cells from umbilical cord bloodUmbilical cord blood is another source of MSCs. These multipotent cells differentiate into mesodermal, endodemal, or ectodermal lineage cells; the latter type is relevant for hypoxia-ischaemia.17–19,22,23 All preclinical trials with these cells have been conducted in rats, using the Rice-Vannucci model.19,43–46 One study reproduces a focal hypoxic-ischaemic episode induced by middle cerebral artery occlusion.19,44 As in the previous case, most studies used the intraventricular route, with a dose of 105 cells.19,43–46 In all trials, this treatment achieved motor and cognitive function recovery by reducing lesion volume and preserving neuronal survival, decreasing levels of markers of microgliosis and astrogliosis.19,43–46 Furthermore, and unlike in the case of MSCs from umbilical cord tissue, these studies report that a greater number of MSCs differentiated to astrocytes and microglia, which may also contribute to the neuroprotective effects of this treatment.19,44,45 It has also been reported that stem cell therapy combined with hypothermia achieves better results than either of the treatments alone, regardless of the timing of treatment onset (6-48 hours after injury).19,43,46 These results show that hypothermia may enhance the neuroprotective effects of stem cell therapy and increase the window period.
Mesenchymal stem cells from placental tissueFew studies have addressed this subject. However, 2 preclinical trials have shown that placental tissue-derived MSCs induce recovery of motor function by reducing inflammation, lipid peroxidation, and oxidative stress, and ultimately neuronal damage.19,47,48 Both trials used the Rice-Vannucci rat model, and cells were administered via the intraventricular route 48 hours after injury, at a dose of 5 × 104 and 106 cells (Table 1).19,47,48
Mesenchymal stem cells from bone marrowBone marrow-derived MSC therapy has been one of the most frequently used approaches.49–51 However, it is no longer used due to the difficulty and low yield of obtaining these cells.49–51 In any case, studies with bone marrow-derived MSCs have provided reliable evidence of the neuroprotective capacity of these cells and their effects on hypoxic-ischaemic injury.19,49–51
Similarly to studies into other types of MSCs, most preclinical trials into bone marrow–derived MSCs have used mice with perinatal hypoxic-ischaemic encephalopathy (Rice-Vannucci model), which were administered at doses from 105 to 2 × 106 cells between the first and the tenth days after injury.19,52–56 In most cases, cells were administered intraventricularly, as this route seems to achieve the greatest cell variability and efficacy.19,53,56 In all studies, treatment with MSCs reduced lesion volume and recovered motor and cognitive function; these effects were more marked when the treatment was administered earlier, in several doses, and at larger doses.19,52–56 In general terms, this effect was achieved by reducing astrogliosis and microgliosis and the subsequent inflammation.19,52–56 Furthermore, treatment with bone marrow MSCs has been reported to achieve greater axonal function, increase the release of trophic factors, and promote the maturation and proliferation of neurons and oligodendrocytes,19,53,57–60 although differentiation into mature neurons has not been observed.19,54 However, animals receiving MSCs combined with hypothermia presented increased infiltration of endothelial and peripheral immune cells, increased release of proinflammatory mediators, and decreased levels of trophic factors.19,61 These results confirm the neuroprotective and neuromodulatory capacity of MSCs when not combined with hypothermia (Table 1).
In conclusion, MSC therapy seems to be useful for preventing hypoxic-ischaemic brain injury due to its neuroprotective properties and its ability to promote cell differentiation, proliferation, and maturation.19,52–60 However, the source of MSCs should be considered: while bone marrow–derived MSCs have been found to have deleterious effects when combined with hypothermia,19,61 the combination of hypothermia and umbilical cord-derived MSC therapy seems to enhance the benefits of both treatments.19,43–46
Endothelial progenitor cellsAnother approach to cell therapy uses endothelial progenitor cells from peripheral blood or bone marrow.62–67 Among many other properties, endothelial progenitor cells express CD34, CD133, and vascular endothelial growth factor receptor.62–67 These markers may play a fundamental role, since they differentially express microRNAs that are involved in the prevention of apoptosis, cytoskeleton remodelling, differentiation into multiple cell lineages, and promoting neovascularisation; this is essential for the treatment of hypoxic-ischaemic brain injury.19,62–67
Rice-Vannucci models have shown that the administration of endothelial progenitor cells 1–2 days after injury improved motor function and decreased lesion volume.19,28,36,68 A single dose of 105 cells administered intraperitoneally achieved motor recovery, in part due to the release of such vasculogenic factors as fibropellin-2 precursor, vascular endothelial growth factor, and insulin-like growth factor 118,19,22 as a result of a phenotypic change to M2 microglia18,19,22,36; this promotes cell maturation and proliferation and regulates cerebral blood flow (Table 1).18,19,22,69,70
Neural stem cellsAfter an ischaemic event, the brain promotes the release of neuronal and glial progenitors to compensate for the neuronal and axonal loss and dysfunction.6,18,71 This function is performed by neural stem cells; these self-renewing multipotent cells are able to differentiate into multiple cell lines, including neurons, astrocytes, and oligodendrocytes.18,72,73 Unfortunately, the release of such proapoptotic factors as calpain and caspase 3 renders the release of neuroblasts and glial progenitors ineffective: approximately 90% of neural progenitor cells will die and the remaining 10% will not be functional.18,74–76 New therapies are being developed to boost self-renewal through the administration of exogenous neural stem cells.18
In preclinical trials, the Rice-Vannucci model is once again the most frequently used.18,77–83 In all cases, animals were administered either 105 or 106 cells, mainly via the intracranial route,18,77–82 although the intranasal route has also been used.18,83 Regardless of the time of treatment onset (from 2 hours to 10 days after injury),18,77–83 administration of neural stem cells reduced neuroinflammation18,78,81,83 and promoted myelination and maturation of oligodendroglial progenitor cells.18,77,80,82,83 This, in turn, promoted synaptic plasticity and axonal growth, stabilised blood-brain barrier permeability, and ultimately reduced apoptosis and lesion volume in the acute and subacute phase, loss of brain tissue in the chronic phase, and motor dysfunction.18,77–82 Furthermore, all these benefits were observed independently of the source of the neural stem cells (embryonic tissue, fetal brain tissue, adult subventricular zone tissue, etc).18,77–82 However, the authors recommend starting treatment no sooner than 24 hours after injury, so that cell viability is not compromised by inflammation and excitotoxicity secondary to brain injury (Table 1).18,78,79,83
In addition to its efficacy, clinical trials conducted at Beijing Hospital (China) have demonstrated the safety of this treatment when administered intrathecally over 2 days in combination with hypothermia (NCT02854579). Therefore, administration of exogenous neural stem cells constitutes a potential treatment for preventing hypoxic-ischaemic brain injury (Table 2).
ConclusionHypoxic-ischaemic brain injury is one of the main causes of CP, the most frequent and severe motor disability in childhood. Injury occurs during the perinatal and postnatal periods, and is characterised by increased excitotoxicity, oxidative stress, and inflammation, which ultimately cause cortical and white matter lesions. Therapeutic hypothermia is the only treatment currently approved for CP; its efficacy is limited, however. Stem cell therapy may provide new opportunities for the treatment of a wide range of disorders, including CP secondary to hypoxia-ischaemia. This treatment reduces inflammation and oxidative stress and promotes cell survival, maturation, and differentiation, which ultimately leads to recovery of motor function. Although most clinical trials have used umbilical cord blood stem cells, some trials have also been conducted with MSCs and neural stem cells, demonstrating their safety in human patients. Furthermore, stem cell therapy has been found to enhance the therapeutic effects of hypothermia. These findings suggest that stem cell therapy may constitute an efficacious strategy for reducing the severity and incidence of CP secondary to hypoxic-ischaemic brain injury.
Author contributionsMV participated in data collection. JMO participated in data collection and reviewed the manuscript. AP participated in data collection, study design, and manuscript drafting.
FundingThis study has received no funding from any public, private, or non-profit organisation.
Conflicts of interestNone.
We wish to thank BioRender for the images provided.