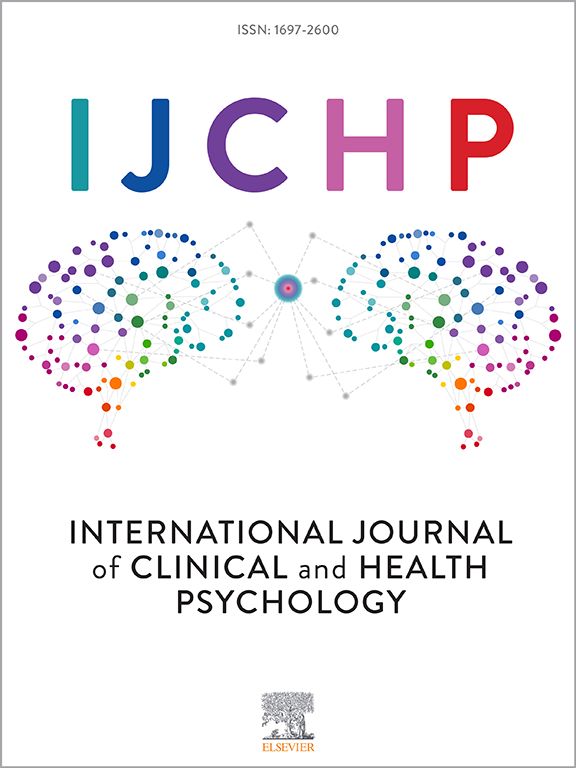
Editado por: Andre R Brunoni, Marie-Anne Vanderhasselt, Leigh Chavert
Más datosThe prefrontal cortex plays a crucial role in cognitive processes, both during anticipatory and reactive modes of cognitive control. Transcranial Direct Current Stimulation (tDCS) can modulate these cognitive resources. However, there is a lack of research exploring the impact of tDCS on emotional material processing in the prefrontal cortex, particularly in regard to proactive and reactive modes of cognitive control. In this study, 35 healthy volunteers underwent both real and sham tDCS applied to the right prefrontal cortex in a counterbalanced order, and then completed the Cued Emotion Control Task (CECT). Pupil dilation, a measure of cognitive resource allocation, and behavioral outcomes, such as reaction time and accuracy, were collected. The results indicate that, as compared to sham stimulation, active right-sided tDCS reduced performance and resource allocation in both proactive and reactive modes of cognitive control. These findings highlight the importance of further research on the effects of tDCS applied to the right prefrontal cortex on cognitive engagement, particularly for clinical trials utilizing the present electrode montage in combination with cognitive interventions.
Cognitive resources are essential to adaptively and flexibly change our actions, emotions and cognitions (Braver, 2012). When dealing with emotional stimuli, the flexible and efficient use of cognitive control resources is vital to override habitual or automatic tendencies. Cognitive control resources are recruited when 1) responding to a stimulus (online or reactive mode of control to resolve conflict after it actually occurs); but also 2) when anticipating a stimulus before the challenging stimulus is presented (proactive mode of control) (De Raedt & Hooley, 2016; Braver, 2012). The former is based on an acute, on-demand corrective mechanism as the need arises, and the latter is based on maintained cognitive resources in order to anticipate upcoming cognitive demands (e.g. (Braver et al., 2009; Vanderhasselt et al., 2014)). Proactive control is expected to be mainly associated with anticipatory and sustained activation of the lateral prefrontal cortex (PFC) (De Raedt & Hooley, 2016), whereas reactive control would entail transient probe-based activity of the same region (in addition to activity in other brain regions), which would reflect detection and/or the resolution of interference only at specific times (Braver, 2012; Vanderhasselt et al., 2009). Research is still ongoing regarding the laterality of PFC activity during both modes of cognitive control, possibly with a role of the left PFC mainly implicated in proactive control whereas the right PFC is involved in both proactive and reactive control (Boudewyn et al., 2020; Pulopulos et al., 2022; but see Gómez-Ariza et al., 2017).
The possibility of augmenting these cognitive abilities has received much attention (e.g., Miniussi et al., 2013). The modulation of neural activity has been found to change prefrontal networks. A promising technique is based on transcranial Direct Current Stimulation (tDCS), a non-invasive neuromodulation that uses constant but weak direct current delivered via at least two electrodes on the scalp and is able to modulate brain neural networks towards an increase or decrease in the endogenous neuronal firing (e.g., Nitsche & Paulus, 2000). Overall, studies report the beneficial effects of tDCS applied to the PFC on general cognitive control processes (Brunoni & Vanderhasselt, 2014; Dedoncker et al., 2016; Feeser et al., 2014). As for the specific influence of tDCS applied to the PFC on both proactive and reactive modes of cognitive control, initial studies have been conducted, providing relatively mixed findings. For instance, Boudewyn et al. (2020) reported a beneficial effect of anodal tDCS applied to the left dorsolateral prefrontal cortex (DLPFC, cathode over the contralateral supraorbital site) on behavioural and electrical markers of proactive control in healthy volunteers. The task was the Dot Pattern Expectancy (DPX) Task, with an index of proactive control but there was no index of reactive control. In contrast, Gomez-Ariza et al. (2017) observed that anodal and cathodal tDCS applied to the left DLPFC (reference electrode placed on the contralateral shoulder) did not modulate performance in specific proactive cognitive control measures of the AXE-continuous performance task (AXE-CPT), which has been extensively used to explore changes in proactive and reactive control. However, it should be mentioned that these authors observed that offline cathodal stimulation over the right DLPFC led participants to be less proactive (or more reactive). In turn, Friehs et al. (2021) recently reported in a systematic review - based on studies in stop-signals tasks - that anodal tDCS over the right prefrontal cortex has the potential to enhance reactive response inhibition, even though neurostimulation may also affect proactive inhibition.
Most tDCS studies investigating proactive and reactive control have been employing variants of the AXE-CPT, where participants must actively maintain cue (context) information and respond to a cue-probe combination on each trial. Proactive control is then conceptualized as a general readiness to perform a task. Yet, it has been proposed that another type of cue-probe design including anticipatory cues instructing one regulation strategy vs. another, specifically before the probe is presented on each trial, with sufficient delays between this cue and the probe would be a promising new design (Kalisch, 2009). In this context, the Cued Emotional Control Task (CECT, Vanderhasselt et al., 2013; Vanderhasselt et al., 2014) is an example of a cue/probe cognitive paradigm. In addition, in this latter task, proactive and reactive modes of control are disentangled in the context of emotional stimuli. In the CECT participants are instructed to respond with the actual or opposite emotion of an upcoming emotional stimulus. Therefore neurophysiological indices can be used during the anticipation period (following the cue), as well as during the target (probe) response period, to quantify the effects of tDCS on proactive and reactive control. Our previous study (Vanderhasselt et al., 2013) showed that modulating the left PFC using tDCS can impact the performance of the abovementioned task, by enhancing reactive control for positive information. To date, a limited number of studies has been conducted to investigate the impact of tDCS applied to the right PFC on proactive and reactive control using a cue/probe design, especially in the context of emotional material. However, this might be interesting, as for instance, according to a recent study (Huang et al., 2017), impulsivity components were correlated with proactive and reactive control, which in turn were associated with brain activity in the right DLPFC, but not within the left DLPFC.
Therefore, the aim of the current within-subjects study was to investigate the effects of anodal tDCS applied to the right DLPFC, compared to sham, on proactive and reactive cognitive control by using the CECT task (Vanderhasselt et al., 2013). Moreover, pupil dilation measure was employed as it is an interesting neurocognitive index to measure cognitive resource allocation (Wel et al., 2018). Pupil dilation is considered a peripheral marker of cortical processing or the intensity of task engagement that can be reliably interpreted in well-controlled contexts (such as luminance). Especially when the dissociation between proactive and reactive control is relevant, pupil dilation is an informative measure. Larger pupil dilation during proactive and reactive modes of control has also been associated with beneficial cognitive performance (Vanderhasselt et al., 2014). Larger pupil dilation compared to baseline is associated with enhanced cognitive performance (Rondeel et al., 2015; van der Meer et al., 2010).
Hence, in the current study, physiological (pupillometry) and behavioral (reaction time in the reactive phase: reaction times, accuracy) indices were measured. We investigated the effects of anodal tDCS applied to the right DLPFC (cathodal over the contralateral supraorbital cortex) on both proactive (anticipation during the cue) and reactive (response during the target) modes of cognitive control. In general, we expected a beneficial effect of active tDCS, as compared to sham, on cognitive control processes, including both proactive (more pupil dilatation) and reactive (more pupil dilatation, higher accuracy and faster reaction times) modes of control. However, given the inconsistent results from previous studies (e.g., Boudewyn et al., 2020; Friehs et al., 2021), we had no clear hypotheses on the differentiation between proactive and reactive control. We expected, based on our previous work, no differences in reactive control for positive or negative stimuli when anodal tDCS is applied to the right DLPFC (Sanchez et al., 2016; Allaert et al., 2019).
Material and methodsThis study was part of a larger project investigating neurocognitive markers of attentional deployment and cognitive control for emotional material (IRB number of the approval of the medical ethical committee of the University Hospital Ghent: 2014/0433).
ParticipantsAn a priori power analysis using G*Power (Faul et al., 2007) for an F-test of a repeated measures design estimated a sample size of 34 to detect with 80% power a moderate effect size (f = 0.25, α = 0.05). A sample of 35 college undergraduates of Ghent University, with an age range from 19 to 40 years (Mean =23.4, standard deviation (SD) =4.43, female= 68.6%), participated in this study. All participants were right-handed, as was an inclusion criteria for participation (no questionnaire was assessed). Based on the Mini International Neuropsychiatric Interview (MINI) (Sheehan et al., 1998) none reported previous neurological or psychiatric disorders. Participants reported having no eye problems or difficulties in vision, even though glasses or contact lenses were allowed. Participants were not pregnant at the time of stimulation and had no metal in or around their scalp.
ProcedureParticipants gave their written informed consent and received a monetary compensation of 40 euros for their participation. The study was conducted in adherence to the Declaration of Helsinki and approved by the medical ethical committee of the University Hospital (2014/0433). The study involved a double-blind, placebo-controlled within-subjects design for each participant receiving real and sham (placebo) stimulation of rDLPFC on two separate days (using a randomized counterbalanced order). After stimulation, participants performed the CECT. Subjective mood ratings were recorded using Visual Analogue Scales (VAS) taken at baseline and after task performance.
Visual analogue scalesIn order to evaluate temporary changes in mood before (Tpre), versus after (Tpost) the CECT paradigm, mood ratings were administered using six visual analogue scales (VAS) providing measures of fatigue, tension, anger, vigor, depression and cheerful (McCormack et al., 1988). Participants were asked to describe how they felt ‘at that moment’ by indicating on horizontal 100 cm lines whether they experienced the five above-mentioned mood states, from ‘totally not’ to ‘very much’. Compound scores of mood were calculated where all the responses to negative and positive (reversed) mood ratings were summed up. The higher the total score, the more negative mood was reported.
Transcranial direct current stimulation (tDCS)Direct electrical current was applied by a saline-soaked pair of surface sponge electrodes (5 × 7 cm, i.e., 35 cm2) and delivered by a battery-driven stimulator (Neuroconn DC-Stimulator, Ilmenau, Germany). To stimulate the right DLPFC, the anode electrode was positioned vertically over the F4 center according to the 10–20 international system for electroencephalogram electrode placement (Klem et al., 1999). The cathode was placed horizontally over the contralateral supraorbital area. This electrode placement and method of DLPFC localization are in accordance with prior tDCS studies (Sanchez et al., 2016; Vanderhasselt et al., 2017). A constant, direct current of 2 mA was applied for 20 min, with 15 s of a ramp up and down at the start and end. For sham stimulation, the electrodes were positioned similarly to when administering active tDCS stimulation; however, the current was ramped up and down 30 s after the start of the session. This procedure is a reliable sham condition (Nitsche et al., 2008; De Smet et al., 2021, 2008).
A sham (placebo)-controlled crossover design was used. On two separate days, participants received 20 min of active or sham stimulation. The order of both stimulation sessions (real tDCS and sham stimulation) was counterbalanced, with an interval of at least 48 h (most participants had an interval of at least 1 week). Fig. 1 shows a visualization of the electric field simulation of the utilized tDCS montage.
shows a visualization of the electric field simulation of the utilized tDCS montage
Note: Computational electric field modeling was performed using the software SimNIBS 4.0 (Puonti et al., 2020). magnE is the magnitude of the electric field plotted in a gray matter surface measured in V/m.
Cued Emotional Control Task (CECT). The CECT (M.-A. Vanderhasselt et al., 2014) was programmed in MATLAB 2012b software (MATLAB, 2012). Eighteen faces (9F/9 M) from the Karolinska Directed Emotional Faces dataset (Lundqvist et al., 2015) were used as stimuli, comprising one frontal happy and one frontal sad expression each (i.e., 36 pictures). Each face was shown in a happy or sad expression (matched for arousal based on the validation (Goeleven et al., 2008). Each trial started with a baseline phase during which a center fixation cross is presented for 2000 ms. Afterwards, one of the two-word cues presented for 500 ms: “actual”, which instructed participants to press a key corresponding to the valence of the emotional expression in the upcoming target face (i.e., press “positive” for an upcoming happy face, press “negative” for an upcoming sad face); and “opposite”, which indicated that participants should make the response corresponding to the opposite valence of the emotional expression in the upcoming target face (i.e., press “negative” for an upcoming happy face, press “positive” for an upcoming sad face). Following the cue word, a cue offset screen was presented for 1500 ms. Afterwards, either a happy or sad face was presented for 2000 ms (target phase). Fig. 2 provides a schematic overview of the different phases during a trial.
Participants completed 20 practice trials using five faces not shown in the experimental blocks, followed by 5 blocks of 36 trials (a total of 180 trials). Each block contained nine trials of each cue/face combination (2 cues x 2 faces), resulting in 36 trials per condition (about 20 min in total). Participants were instructed to respond as quickly and accurately as possible immediately after the face presentation, even though the face stayed on the screen after the response was made. The assignment of labels (happy-sad) to the two buttons in the response box was counterbalanced across participants.
Pre-processing of behavioral dataGiven the skewed, asymmetric, non-normal distribution of RTs, outlier data points were identified using the Double Median Absolute Deviation (Leys et al., 2013), which is a robust outlier detection method that is less dependent on the properties of the data distribution. Outlier sensitivity was set to the default scale factor k = 3, and identified outlier data points were disregarded from analyses on RTs (5.27%).1
PupillometryPupillometry was carried out using a High-Speed Video Eye Tracker Toolbox system (Cambridge Research Systems, UK) at a sample rate of 250 Hz. For optimal measurements, participants were comfortably seated approximately 75 cm from the eye tracker (resulting in a visual angle of approximately 5.7° between the picture's center and the screen's center) and the eye tracker was calibrated using a standard nine-point calibration sequence. Pupil data were sent digitally from the eye tracker to a computer, along with event markers indicating the beginning and end of each phase (baseline, cue, target) in all trials. The average luminance of the monitor was 104 cd/m2 (4002 Td) and the output of the display was gamma corrected. Also, the light in the room was kept as constant as possible, by using a dimmer light. All pictures were gray-scaled.
Pre-processing of pupillary dataThe data were pre-processed in PhysioData Toolbox 0.6.3 (Sjak-Shie, 2022). First, 3 frequently occurring artifacts, including those produced by eye-blinks (dilation speed outliers and edge artifacts, trend-line deviation outliers, and temporally isolated samples) were removed using a 4-pass deviation filter. Next, the pupil data of the left and right eye were aggregated, resulting in mean pupil size time series data. To increase the temporal resolution and smoothness of the data, the signal was upsampled (1000 Hz) and interpolated. The signal was not interpolated over gaps of missing data that were larger than 250 ms. The resulting signal was then smoothed using a zero-phase low-pass filter set to 4 Hz. Then, for every trial, 3 epochs (baseline, cue,2 target), each of 2 s long were defined, and pupillary responses3 during the cue and target phase were calculated by subtracting the average pupil size during the baseline from the pupil time series signal during the cue and target phase, respectively. The smoothed epoched time series data was then downsampled to 50 Hz to preserve computational resources for the subsequent analyses. Epochs containing 50% or more missing data were disregarded from analyses (17.61%).
Data analytic planThe data was analyzed in R 4.0.2 (Team & Others, 2013) in conjunction with RStudio 1.3.1056. Analyses were performed using (generalized) linear mixed models ([G]LMMs) fitted via the ‘lmer’ and ‘glmer’ functions of the ‘lme4’ R package (Bates et al., 2015). The statistical significance level was set to p < .05 and p-values for the fixed effects were estimated with the ‘lmerTest’ R package, using the Satterthwaite approximations to degrees of freedom (Kuznetsova et al., 2017). The sum of squares was calculated using the type III approach and the analysis-of-variance (ANOVA) tables for each (G)LMM were computed using the ‘anova’ R function (for LMM) or the ‘Anova’ function of the ‘car’ R package (for GLMM; (Fox & Weisberg, 2018)). Observed significant effects in the ANOVA tables were followed-up by pairwise comparisons of the estimated marginal means (EMMs) or pairwise comparisons of the EMMs of linear trends (i.e., comparisons of slopes), via the ‘emmeans’ and ‘emtrends’ function in the ’emmeans’ R package.
To investigate the effect of tDCS on mood, a LMM featuring the compound score for negative mood as dependent variable, tDCS (active, sham) and time (pre, post) as fixed factors, and subject as random intercept (Negative mood ∼ tDCS * Time + Session + (1|Subject)).
To investigate the effect of tDCS on reaction times (RTs), in line with the recommendations by Lo and Andrews, (2015) a series of (G)LMMs were compared to assess the model that best satisfied the statistical assumptions underlying the model (see supplementary materials). A GLMM of the gamma distribution family with an identity link function was retained. This GLMM featured reaction time as dependent variable, tDCS (active, sham), emotion (happy, sad), and cue (actual, opposite) as fixed factors, session (0, 1) as control variable, and subject as random intercept (RT ∼ tDCS * Emotion * Cue + Session + (1|Subject)). For the analysis of RTs, only RTs during correct trials were considered.
To investigate the effects of tDCS on accuracy, a GLMM of the binomial family with a logit link was employed (Dixon, 2008). This GLMM featured accuracy as dependent variable, tDCS (active, sham), cue (actual, opposite), emotion (happy, sad) as fixed factors, session (0, 1) as control variable, and subject as random intercept (Accuracy ∼ tDCS * Emotion * Cue + Session + (1|Subject)).
To investigate the effect of tDCS on pupillary responses and baseline pupil size, 3 LMMs were fitted with a) average baseline pupil size (Average baseline pupil size ∼ tDCS + Session + (1|Subject)), b) average pupillary response during the cue phase (Averaged cue pupillary response ∼ tDCS * Cue + Session + (1|Subject)), and c) average pupillary response during the target phase (Averaged target pupillary response ∼ tDCS * Cue * Emotion + Session + (1|Subject)), as dependent variables, respectively. All these LMMs featured tDCS (active, sham) as fixed factor, session (0, 1) as control variable, and subject as random intercept. Furthermore, the LMM during the cue and target additionally featured cue (actual, opposite) as fixed effect, whereas the LMM during target also featured emotion (happy, sad) as fixed effect. Finally, to assess the time windows of when significant effects of tDCS on each averaged pupillometric index occur (i.e., baseline pupil size, pupillary response during cue, pupillary response during target), cluster-based permutation tests for linear mixed models were performed on the non-averaged time series pupil data using the ‘clusterperm.lmer’ function of the ‘permutes’ R-package. The permutation tests for each of the 3 pupillometric indices was based on the respective LMM formula of each index, with the addition of time (0 - 2) as a continuous time series variable. Using this approach (Maris & Oostenveld, 2007), family-wise type I error was controlled for multiple comparisons using a non-parametric cluster thresholding method based on 1000 permutation samples. Permuted likelihood-ratio tests were used to compute cluster-mass statistics.
For the analysis of mood and pupillometric data, analysis of variances were computed using type III F-tests, reporting the F-test statistic, degrees of freedom in the numerator and denominator, and p-value. Follow-up tests report the raw unstandardized coefficient (b), the standard error (SE), the t-test statistic, and the p-value. For the analysis of RTs and accuracy, analysis of variances were computed using type III Wald chi square tests, reporting the chi square test statistic (χ2), degree of freedom and the p-value. For RTs, follow-up tests report the raw unstandardized coefficient (b), the standard error (SE), the z-test statistic, and the p-value. For accuracy, follow-up tests report the odds ratio (OR), the 95% confidence interval (95% CI), the z-test statistic, and p-value.
ResultsOverall, 20% of all participants correctly indicated when they received real and sham stimulation (first versus second session). Most of the volunteers were forced to make a guess. Overall, the stimulation blinding was successful.
MoodThe linear mixed model for negative mood showed a significant effect of Time, F(1, 100) = 56.76, p < .001, revealing that negative mood increased significantly after as compared to before (active and sham) stimulation, b = 7.29, SE = 0.97, t = 7.53, p < .001. Yet, the main effect of Stimulation, nor the interaction between both, were not significant (all Fs < 1.04, all Ps > 0.31). The rest-rest reliability for self-reported negative mood was acceptable, r = 0.70, p < .001
Behavioral dataReaction timesThe GLMM for RTs showed a significant effect of tDCS, χ2(1) = 9.77, p = .002, cue, χ2(1) = 81.63, p < .001, session, χ2(1) = 236.11, p < .001, and cue × emotion, χ2(1) = 15.81, p < .001. The effect of tDCS showed that active (versus sham) tDCS was associated with slower RTs, b = 13.2, SE = 4.24, z = 3.13, p = .002 (see Fig. 3A). The effect of session showed that RTs were faster during the second (versus first) session, b = 67.2, SE = 4.37, z = 15.37, p < .001. Decomposition of the cue × emotion interaction showed that happy (versus sad) emotions reduced RTs when the cue was actual, b = −12.7, SE = 5.35, z = 2.37, p = .02, whereas happy (versus sad) emotions increased RTs when the cue was opposite, b = 18.3, SE = 5291, z = 3.46, p = .001. All other effects (i.e., emotion, cue × tDCS, emotion × tDCS, cue × emotion × tDCS) were non-significant (all χ2 < 1.38, all Ps > 0.24). The test-retest reliability for RTs was acceptable, r = 0.74, p < .001.
AccuracyThe GLMM for accuracy data showed a significant effect of tDCS, χ2(1) = 5.85, p = .02, session, χ2(1) = 4.27, p = .03, and cue × emotion, χ2(1) = 7.75, p = .01. The effect of tDCS showed a significant decrease in the odds of providing a correct response during active (versus sham) tDCS, OR = 0.85, 95% CI (0.75, 0.97), z = 2.42, p = .02 (see Fig. 3B). The effect of session showed that the odds of a correct response were higher during the first (versus second) session, OR = 1.15, 95% CI (1.01, 1.30), z = 2.07, p = .04. Decomposition of the cue × emotion interaction showed that happy (versus sad) emotions increased the odds of providing a correct response when the cue was actual, OR = 1.29, 95% CI (1.07, 1.55), z = 2.67, p = .01, whereas when the cue was opposite, happy (versus sad) emotions were not associated with a significant difference in the odds of a correct response, OR = 0.89, 95% CI (0.75, 1.07), z = 1.25, p = .21. All other effects (i.e., emotion, cue, cue × tDCS, emotion × tDCS, cue × emotion × tDCS) were non-significant (all χ2s < 2.03, all Ps > 15). The rest-retest reliability for accuracy was acceptable, r = 0.77, p < .001
Pupillary responsesBaselineThe LMM for average pupil size during the baseline period showed a significant effect of tDCS, F(1, 10,519) = 8.83, p = .003, with smaller pupil size during active (versus sham) tDCS, b = −0.01, SE = 0.004, t = −2.97, p = .003. In addition, an effect of session was present, F(1, 10,519) = 210.54, p < .001, with larger pupil size during the second (versus first) session, b = −0.05, SE = 0.004, t = −14.51, p < .001. Follow-up cluster-based permutation tests showed that the effect of active (versus sham) tDCS occurred between 1 and 2 s during the baseline epoch (see Fig. 4). The test-retest reliability for baseline pupil size was good, r = 0.88, p < .001.
Effects of tDCS on baseline pupil size and pupillary responses during cue and target. For pupillary responses during cue and target, values above the dashed line indicate an increase in pupil size relative to baseline (i.e., pupil dilation), whereas values below indicate a decrease in pupil size (i.e., pupil constriction). The area highlighted in gray represents the time window in which significant differences emerged based on cluster-based permutation tests.
The LMM for average pupillary responses during cue presentation showed a significant effect of tDCS, F(1, 9565.3) = 12.48, p < .001, with larger pupil constriction occurring during active (versus sham) tDCS, b = −0.01, SE = 0.003, t = −3.53, p = .0004. All other effects (i.e., cue, tDCS × cue, session) were non-significant (all Fs < 3.38, all Ps > 0.07). Follow-up cluster-based permutation tests showed that the effect of active (versus sham) tDCS occurred throughout the whole cue epoch, from 0 to 2 s (see Fig. 4). The test-retest reliability for pupillary reactivity during cue was good, r = 0.84, p < .001.
TargetThe LMM for average pupillary responses during target presentation showed a significant effect of tDCS, F(1, 10,516) = 5.63, p = .02, with smaller pupil dilation occurring during active (versus sham) tDCS, b = −0.01, SE = 0.003, t = −2.37, p = .02. In addition, an effect of session was observed, F(1, 10,517) = 29.99, p < .001, with larger pupil dilation occurring during the second (versus first) session, b = −0.02, SE = 0.003, t = −5.48, p < .001. All other effects (i.e., cue, emotion, cue × emotion, cue × tDCS, emotion × tDCS, cue × emotion × tDCS) were non-significant (all Fs < 1.05, all Ps > 0.31). Follow-up cluster-based permutation tests showed that the effect of active (versus sham) tDCS occurred between 0 and 1 s during the target epoch (see Fig. 4). The test-retest reliability for pupillary reactivity during target was good, r = 0.81, p < .001.
DiscussionThe present sham-controlled within-subjects study aimed to examine the influence of anodal transcranial direct current stimulation (tDCS) applied to the right prefrontal cortex (with the cathode positioned at the contralateral supraorbital site) on cognitive resources during proactive and reactive modes of cognitive control in the context of emotional stimuli. Pupil dilation, a reliable indicator of cognitive resource allocation during task performance, was monitored throughout the cognitive paradigm, the cued emotional control task (CECT). Our offline tDCS design was found to be adequately blinded. The results indicated that negative mood increased following as compared to before the cognitive task, but this increase was not significantly different between the real and sham stimulation conditions. This implies that the observed effects on cognition can be attributed to tDCS and not to changes in mood. The findings of the present study, evidenced by both behavioral and physiological measures, showed a reduction in both proactive (based on the pupil size) and reactive modes (based on RT, accuracy and pupil size) of cognitive control with active tDCS compared to sham stimulation applied to the right prefrontal cortex. These results will be discussed in detail in the following paragraphs.
Results indicated that, as compared to sham stimulation, active tDCS resulted in a decrease in behavioral performance during reactive control, as evidenced by longer reaction times on correct trials and lower overall accuracy across all trials during the CECT. Despite the relatively straightforward nature of the task, which is known to be susceptible to learning effects with repeated administration, tDCS reduced behavioral performance while controlling for session order. Moreover, the results of pupil dilation also supported the findings of the behavioral data, demonstrating smaller changes in pupil size during the cue and target phases of the CECT with real tDCS compared to sham stimulation. These observations were independent of trial type, both during the cue and probe phases. These results suggest that, following real tDCS, healthy individuals allocate fewer cognitive resources during both proactive and reactive phases of the CECT. Additionally, smaller pupil size was observed during the baseline period at the beginning of the trial in the real tDCS (as compared to the sham) condition. Smaller pupil size has been linked to low vigilance, sleepiness, and decreased engagement in the task (Zénon, 2019). These findings suggest that, compared to sham, healthy volunteers were less engaged in the task following active real tDCS over the right prefrontal cortex.
All in all, the present study results - both behavioral and pupil data - revealed a reduction in cognitive resource allocation following tDCS applied to the right prefrontal cortex as compared to sham stimulation during the CECT. This decrease in resource allocation suggests that participants were less engaged and less cognitively active during the task. Furthermore, this effect was independent of the emotional valence of the stimuli, or phase of the trial (baseline, cue or target), indicating a general effect of tDCS during the CECT. Although it could be argued that the task was perceived as boring or that participants were not motivated to perform the task, the observation of increased pupil dilation during the second session of the CECT (independent of real or sham stimulation) suggests that the task itself was not the cause of reduced resource allocation. Rather, the reduced cognitive performance observed in this study appears to be specifically related to active tDCS applied to the right prefrontal cortex.
The unexpected finding of reduced behavioral performance following tDCS applied to the right PFC in our study raises several questions. One key observation from Friehs et al.'s systematic review (Friehs et al., 2021) is that most successful trials using anodal tDCS over the right PFC for reactive control employed an extracephalic cathode. It is possible that, in our study, the use of both electrodes placed on the frontal area of the participant's head resulted in reduced PFC activation. Alternatively, an extracephalic electrode may be necessary to activate deeper brain regions related to the outcome, although this would require further examination through neuro-imaging techniques (fMRI/EEG). Another explanation, based on electric field simulations, is that other areas of the PFC were targeted following tDCS, such as the medial PFC. It has been shown that positioning large tDCS electrodes (35cm²) bilaterally (F3/F4 or F4/Fp1) may not maximally target the DLPFC per se, but that hat other parts of the PFC may receive stronger electric field strengths (article in preprint: Soleimani et al., 2022). In addition, future research should also explore the potential impact of laterality in tDCS montage (left vs. right) and their electric field simulation patterns on different forms of cognitive performance during the CECT. More research is warranted to understand why tDCS applied to the left PFC results in an emotion-specific effect in reactive control (Vanderhasselt et al., 2013), whereas this is not the case following tDCS applied to the right PFC. Additionally, further examination of stimulation parameters that may exhibit non-linear effects (Weller et al., 2020) is of interest.
The current study provides insights into the implications of neuromodulation interventions in clinical and health psychology. The use of tDCS with an anode placed over the right PFC has been employed for alcohol addiction or craving protocols (Fregni et al., 2021) and attention deficit hyperactivity disorder (Leffa et al., 2022). Typically, tDCS is combined with cognitive interventions to enhance its effects (Dedoncker et al., 2021). These cognitive interventions require varying types of cognitive control processes, which are crucial mechanisms underlying behavior and cognitive performance. Our results indicate that a single session of tDCS applied to the right DLPFC might lead to reduced cognitive engagement during both proactive and reactive control and baseline, which may hinder the modulation of behavior such as decision-making and approach-avoidance. Further research is necessary to investigate this effect and to determine if there are differences between clinical and healthy samples, and what kind of cognitive paradigms reduce cognitive engagement.
The study has some strengths, such as the combination of pupil dilation and behavioral indices using a well-powered within-subject design. However, there are some limitations, including the confounding of sex in the cue presentation due to a programming error and the limited generalizability of the results to the wider population as all volunteers were young undergraduates. In addition, the high percentage of female participants (70%) in the study may have biased the results towards increased performance, even though more research is necessary to test the effects of gender. Additionally, the effects were measured immediately following a single session of tDCS, and the effects of multiple sessions and longer follow-up periods are still unknown. Finally, even though we observed no effects on mood that were different between both real and sham stimulation, we did not systematically evaluate discomfort/adverse effects of tDCS (see Antal et al., 2017). Even though we don't expect a big discomfort, mild tDCS adverse effects may have impacted the results.
In conclusion, results from the study suggest that tDCS applied to the right PFC reduced resource allocation and impaired cognitive performance during proactive and reactive control modes. Although more research is necessary, these findings have implications for clinical trials using similar electrode montages in combination with cognitive interventions to develop non-pharmacological interventions in clinical and health psychology.
Analyses in which these outlier data points were included are presented in supplementary materials.
The epoch for the cue consisted of both the screen showing the cue as well as the cue offset screen.